Epifaunal Foraminifera in an Infaunal World: Insights Into the Influence of Heterogeneity on the Benthic Ecology of Oxygen-Poor, Deep-Sea Habitats
- 1Department of Earth and Environmental Systems, 159 Science Bldg, Indiana State University, Terre Haute, IN, United States
- 2Integrative Oceanography Division, Scripps Institution of Oceanography, San Diego, CA, United States
- 3Marine Environmental Biology, Department of Biological Sciences, University of Southern California, Los Angeles, CA, United States
A reduction in dissolved oxygen availability in marine habitats is among the predicted consequences of increasing global temperatures. An understanding of past oxygenation is critical for predictions of future changes in the extent and distribution of oxygen minimum zones (OMZs). Benthic foraminifera have been used to assess changes in paleo-oxygenation, and according to prevailing thought, oxygen-poor marine benthic habitats are dominated by sediment-dwelling infaunal foraminifera, while more oxygenated environments are populated with more epifaunal taxa. However, in this study we found elevated densities of epifaunal taxa in oxygen-poor habitats. A series of 16 multicores were taken on depth transects (360–3000 m) across an OMZ in the Southern California Bight to investigate the ecology of living (rose bengal stained) benthic foraminifera. Dissolved oxygen concentrations in bottom water at sampling sites varied from 21 to 162 μmol/l. Sampling focused on bathymetric highs in an effort to collect seafloor surface materials with coarse sediments in areas not typically targeted for sampling. Mean grain size varied from about 131 (gravelly sand) to about 830 μm (coarse sand with fine gravel). Vertical distribution patterns (0–2 cm) were consistent with those of conspecifics reported elsewhere, and reconfirm that Cibicidoides wuellerstorfi and Hanzawaia nipponica have a living preference at or near the sediment-water interface. As expected, assemblages were dominated by infaunal taxa, such as Uvigerina and Bolivina, traditionally associated with the supersaturated, unconsolidated mud, characteristic of OMZ habitats, suggesting that these taxa are not sensitive to substrate type. However, despite dysoxic conditions (21–28 μmol/l), epifaunal taxa comprised as much as 36% of the stained population at the five sites with the coarsest mean grain size, while other measured environmental parameters remained relatively constant. We suggest that these epifaunal taxa, including C. wuellerstorfi, prefer habitats with coarse grains that allow them to remain at or above the sediment-water interface. These results suggest that seafloor habitat heterogeneity contributes to the distribution of benthic foraminifera, including in low-oxygen environments. We submit that paleo-oxygenation methods that use epifaunal indicator taxa need to reconsider the dissolved oxygen requirements of epifaunal taxa.
Introduction
Geographic and bathymetric variability in deep ocean oxygen concentrations has a profound impact on marine benthic ecosystem distribution patterns in modern and ancient oceans. As global temperatures rise, areas of oxygen-poor environments, including oxygen minimum zones (OMZs) are predicted to increase, changing seafloor habitats and species distributions (Levin, 2003). Understanding the responses of benthic organisms to changes in oxygen variability is the key to both predicting changes in benthic ecosystem distribution patterns of a deoxygenated future and interpreting the fossil record of dissolved oxygen changes of the geologic past.
As a result of their ecological and biogeochemical sensitivity to changes in their habitat in modern environments, benthic foraminifera preserved in the fossil record are often used as indicators of paleoenvironmental changes (e.g., Murray and Bowser, 2000). The utility of fossil benthic foraminifera in assessments of ancient changes in ocean conditions relies upon an understanding of the factors that influence modern foraminifera. As new information from living populations becomes available, methods using benthic foraminiferal proxies are modified to improve interpretations of the fossil record. For example, the realization that different benthic foraminifera have different microhabitat preferences (Corliss, 1985), in which some species live at or above the sediment-water interface (epifauna) while others live within the sediments (infaunal), dramatically changed the way that fossil foraminifera were used to evaluate paleoceanographic conditions (e.g., McCorkle et al., 1990; Jorissen et al., 2007).
One of the methods used to assess ecological and physiological limitations of deep-sea benthic foraminifera has been to examine species distributions from available core-tops and compare these with environmental parameters (reviewed in Murray and Bowser, 2000). Through this method and other techniques, the ecology of many benthic foraminiferal species has been characterized and applied in paleoenvironmental proxy analyses. Calcareous benthic foraminifera are often abundant in organic-rich, oxygen-poor environments in the modern ocean (reviewed in Sen Gupta and Machain-Castillo, 1993; and Bernhard and Sen Gupta, 1999). Foraminiferal studies of organic-rich, oxygen-poor environments have been typically based on samples from centers of oxygen-stressed basins, with characteristically thixotropic sediments resulting from organic flux generated by high surface productivity. Based largely on the abundance and rarity of different foraminiferal species in these organic-rich, oxygen-poor settings compared with other regions, ecological preferences and environmental limitations have been inferred and applied in proxy methods to reconstruct changes in both productivity (e.g., Loubere and Fariduddin, 1999) and paleoxygenation (Kaiho, 1991, 1994, 1999; Jannink et al., 2001; Schmiedl et al., 2003; reviewed in Jorissen et al., 2007). Many studies that use foraminifera to evaluate bottom water oxygen (BWO) concentrations consider epifaunal species as indicators of well-oxygenated environments (reviewed in Jorissen et al., 2007). For example, Kaiho (1991) proposed, and later modified (Kaiho, 1994, 1999), the Benthic Foraminiferal Oxygen Index (BFOI), where benthic foraminiferal species are grouped into oxygenic indicator categories (i.e., oxic, suboxic, and dysoxic). Species of Cibicidoides, in particular, along with several other epifaunal taxa, are regarded in BFOI analyses as “oxic indicator species,” occurring in abundance where BWO values are >67 μmol/l (Kaiho, 1991, 1994, 1999). Likewise, deep infaunal taxa which typically dominate organic-rich, oxygen-poor environments, are regarded as indicators of oxygen-poor environments (Bernhard, 1986; Sen Gupta and Machain-Castillo, 1993; Bernhard and Sen Gupta, 1999, reviewed in Jorissen et al., 2007). BWO concentrations and organic input (food availability) are considered primary factors influencing benthic foraminiferal distribution and abundance (e.g., Perez-Cruz and Machain-Castillo, 1990; Rathburn and Corliss, 1994; Jorissen et al., 1995, 2007; Koho et al., 2008). However, relatively few studies have evaluated protoplasm-containing foraminifera from coarse-grained habitats in oxygen-poor settings. Substrate and attachment surfaces are known to be important for epifaunal foraminifera (e.g., Lutze and Thiel, 1989; Burkett et al., 2016), and increased abundances of epifaunal taxa in coarser-grained sediments has been noted in deep-water environments (Schönfeld, 2002). An understanding of the factors that influence benthic foraminifera in oxygen-poor environments is needed for high-resolution paleoceanographic studies (e.g., Stott et al., 1996; Hendy and Kennett, 2000), and to predict the responses of benthic species to deoxygenation in a globally warmer future.
Here we present results of living (rose bengal stained) benthic foraminifera from a depth transect, targeting coarse-grain sediment habitats, across a range of dissolved BWO concentrations in the Southern California Bight (SCB). Specific objectives of this research were to:
1. Examine living (rose bengal stained) benthic foraminiferal distribution patterns in the SCB.
2. Assess the ecology of foraminiferal species living in oxygen-deficient habitats of the SCB.
3. Evaluate paleoceanographic implications of the study’s major findings.
Results from this study provide information relevant to both the ecology of modern oxygen-deficient seafloor habitats and fossil based assessments of paleoceanographic changes.
Materials and Methods
Regional Setting
The Southern California Bight (Figure 1) extends from Point Conception to just south of San Diego. Oceanographically the region is dominated by the California Countercurrent, formed by a large-scale eddy in the California Current, along with seasonal upwelling resulting from strong offshore winds (Nelson et al., 1987). Most of the seafloor in this region is characterized by deep basins, separated by ridges, sills, and islands that run parallel to the shore. Together, these features have been referred to as “The Southern California Borderland” (Emery, 1960). California Borderland basin sediments are typically comprised of thixotropic, organic-rich, mud to silt-sized material on the basin floor, with coarse sediments dominating the bathymetric highs surrounding each basin (Gorsline et al., 1984). This region has been monitored by the California Cooperative Oceanic Fisheries Investigations (CalCOFI), collecting biologic and hydrographic data from a consistent array of stations since 1950. Typical data collected down to 500 m depth include temperature, salinity, oxygen, phosphate, silicate, nitrate and nitrite, chlorophyll, 14C, primary productivity, phytoplankton biodiversity, zooplankton biomass, and zooplankton biodiversity (Bograd and Lynn, 2003). Seafloor samples for our study were collected from the SCB in July, 2011. CalCOFI reports indicate that upwelling off of central and southern California resumed earlier and stronger in the spring of 2010 after recovery from El Niño, which was in turn responsible for regional variability that persisted through the 2010–2011 winter. As a result of strong upwelling, robust sea surface productivity was evident within the SCB during the Summer of 2011 (BjorkstEdt et al., 2011). The OMZ of this region typically resides between 100 and 900 m water depth, with minimum oxygen values occurring from 300 to 500 m (Karstensen et al., 2008), though we found our lowest oxygen concentrations at our 1000 m site.
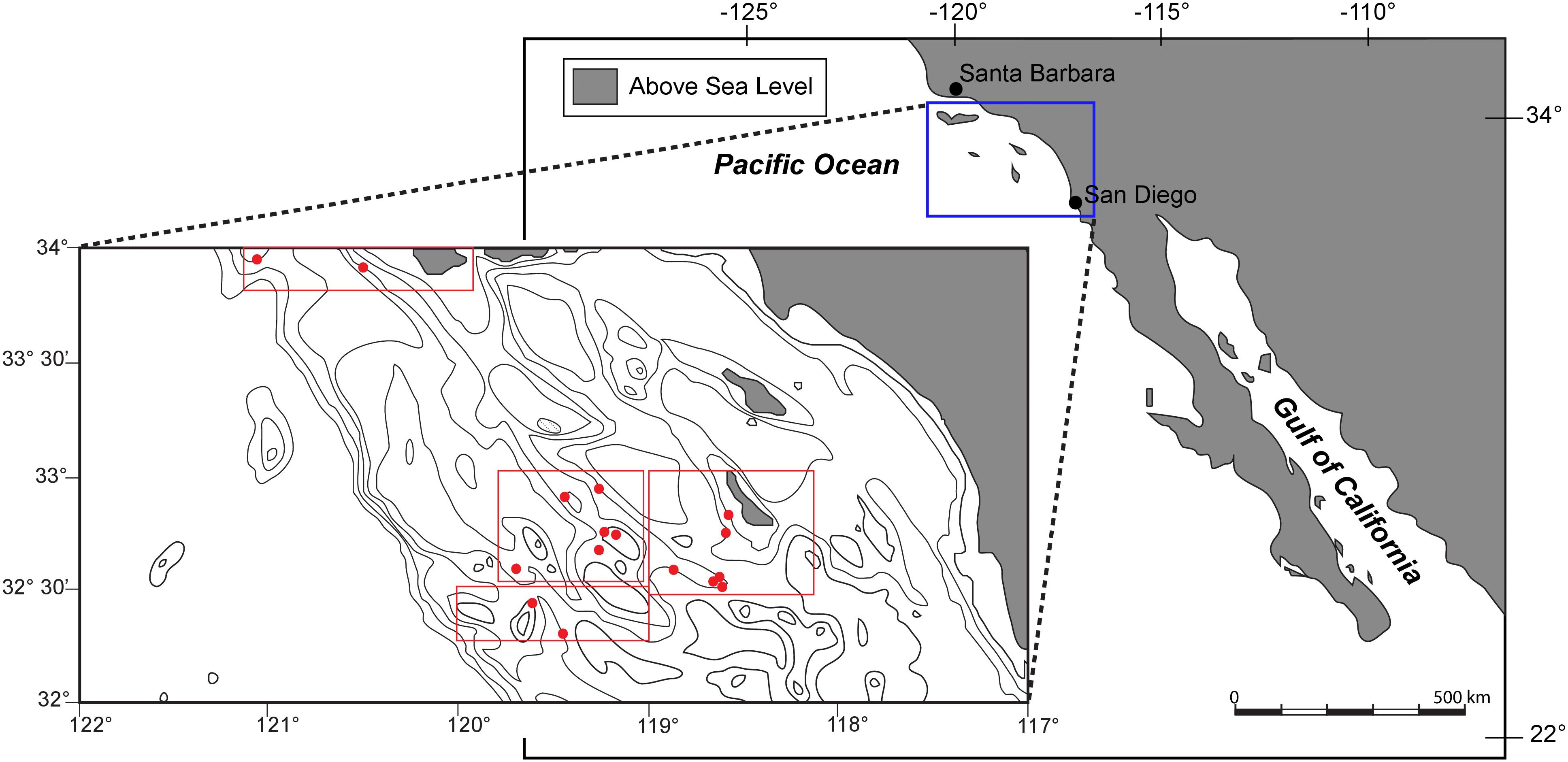
FIGURE 1. Map and bathymetric chart of the study area within the Southern California Bight. Multicore locations, labeled with red filled circles, are described in detail with depth and environmental parameters in table one.
Sample Collection
Samples were collected with an Ocean Instruments multicorer during an R/V New Horizon cruise (NH1108 July 15–21, 2011). Within the study area, edges of basins and bathymetric highs were targeted in an effort to examine the distribution of living foraminiferal assemblages in oxygen-poor habitats with coarse-grained sediments. Sites were sampled from water depths of 360–2969 m, with BWO values ranging from 21 to 162 μmol/l (Table 1). The top 0–1 cm interval was sampled, then cores were sectioned into half-centimeter intervals (i.e., 0–1, 1–1.5, 1.5–2, etc.) and preserved in a 4% buffered formaldehyde solution (37% formaldehyde buffered with Mule Team Borax©), following methods outlined in Rathburn and Corliss (1994).
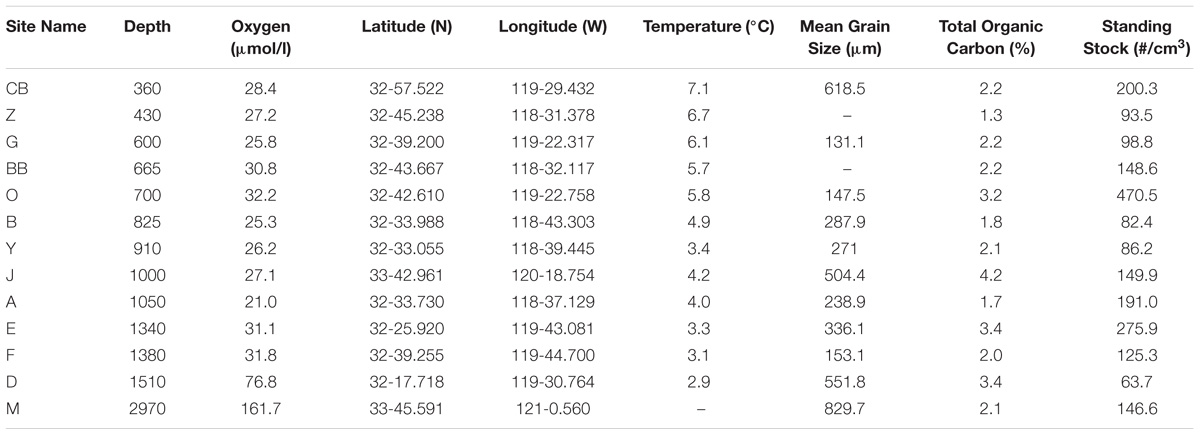
TABLE 1. Core locations, depths, environmental parameters (oxygen, temperature), habitat parameters, (substrate mean grain size and total organic carbon), and live foraminiferal abundances.
Surface sediments (0–1 cm) were also collected from twelve core locations and preserved by freezing (−80°C) for total organic carbon (TOC) and particle size analysis. Upon recovery on the ship, cores selected for microprofiling of dissolved oxygen were immediately moved to a controlled temperature environment (4°C) onboard the ship. The cores were subsequently sectioned into 0.5 and 1 cm intervals under a nitrogen atmosphere for pore water analyses.
Oxygen Microprofiles
Microprofiles of oxygen concentration were measured in vertical intervals of 250 mm using Clark-type amperometric oxygen sensors (Unisense, Aarhus Denmark) using methods outlined in Revsbech and Jørgensen (1986) and Revsbech et al. (1989). BWO for five of the 13 core sites were obtained from microprofiles (sites at 665, 700, 1000, 1510, and 2970 m). Sensors were attached to computer controlled motorized micromanipulators and driven vertically into the sediments on μm to mm intervals. Signals were amplified and transformed to millivolt (mV) by a 2-channel picoammeter (PA 2000; Unisense, Aarhus Denmark) and directly recorded on a computer using the software Profix (PyroScience, Aachen Germany). A linear calibration was performed on board immediately prior to measurements at 0% and 100% oxygen saturation. Calibration chambers filled with filtered seawater were kept at in situ temperature and purged by nitrogen or bubbled with air to create the respective standards.
Bottom Water Properties
Bottom water dissolved oxygen, temperature, and salinity values were obtained from CTD casts (rosette-equipped with an oxygen sensor) at eight of the 13 core sites. The remaining five core site BWO values were determined by oxygen microprofiling as mentioned above.
Sediment Properties: Particle Size and Total Organic Carbon
Both laser diffraction particle-size analysis and conventional dry sieving were used to determine grain size distribution for the surface sediments (0–1 cm) at the locations where surface sediments were available. Coarse-grained substrates led to difficulties in collecting multicores, and in a few locations the only cores available were used for foraminiferal analyses, with no sample remaining for particle size. Samples were analyzed at the National Lacustrine Core Facility (LacCore) on a Partica LA-950 Laser Diffraction Particle Size Distribution Analyzer to capture high-resolution data on the mud to sand size fraction (0.022–2000 μm). To analyze the sand and gravel portion, sediment samples were dry sieved on a ¼ phi interval from 5 (31 μm) to −2 (4 μm). Sediments were removed from sieves and weighed. All data (weight %) were entered into GRADISTAT (Blott and Pye, 2001) to generate statistical and graphical outputs including the mean and sorting of each sample. Surficial sediment samples for each of the multicores were sent to the University of Florida for analyses of organic carbon using a Carlo Erba NA1500 CNHS elemental analyzer.
Sample Processing
Rose bengal (65 mL) was added to each sample and allowed to stain for a minimum of 7 days. The limitations of rose bengal stain are well-known (Bernhard et al., 2006), and using a conservative approach, considering only those individuals with multiple, brightly stained chambers of protoplasm as alive at the time of collection, rose bengal staining provides a reliable assessment of living assemblages (Altenbach and Sarnthein, 1989; Murray and Bowser, 2000).
In order to determine original sediment volumes for each sample and to standardize for comparison between samples, volumetric procedures were followed as outlined in Rathburn and Corliss (1994). Cores were wet sieved from 0 to 2 cm (0–1, 1–1.5, and 1.5–2 cm), over a 63 and 150 μm mesh sieve. Using a modified Otto microsplitter (Otto, 1933), both the >150 μm and 63–150 μm portions were wet split to feasible working sizes, with a minimum target of 300 specimens in the >150 μm portion and 100 specimens in the 63–150 μm portion, and picked for living (stained) benthic foraminifera. Picked specimens were placed on microslides, identified to species, and counted.
Faunal distribution of the >150 μm fraction were analyzed in the surface sediments for each sampling location across the depth/oxygen transect. In a low oxygen environment, the majority (∼90%) of living benthic foraminifera typically inhabit the top 1 cm of sediment (Bernhard and Sen Gupta, 1999). Standing stocks of calcareous and agglutinated taxa were calculated for each site, and presented as number of individual foraminifera per area (#foraminifera/50 cm2).
Diversity indices, including species richness (S), Shannon–Wiener diversity index (H), and evenness (J) were calculated using the free statistical software PAST (PAleontological STatistics; Version 3.05; Hammer et al., 2001).
For these analyses:
Richness (S) = count of number of taxa per site
Diversity (H) =
Evenness (J) = H/Hmax = H/lnS
With pi representing the proportion of the individual taxon to the population.
Results
Bottom Water Properties
Bottom water oxygen values examined in this study ranged from 21 to 161 μmol/l (Figure 2). The terminology of Bernhard and Sen Gupta (1999) will be used, where “anoxic” refers to those regions where BWO equals 0 μmol/l, “dysoxic” refers to BWO between 4.5 and 45 μmol/l, and “oxic” represents BWO greater than 45 μmol/l. Site A (1000 m) is the only core site along the transect where dissolved BWO of 21 μmol/l was below 22.3 μmol/l and within the OMZ as defined by Helly and Levin (2004). The majority of core sites along the transect had dysoxic BWO values that ranged from 22 to 45 μmol/l. Core sites in the dysoxic zone included those at 360, 429, 600, 665, 700, 826, 910, 994, 1339, and 1378 m. Two sites, at 1510 and 2969 m, occur in water depths below the OMZ where BWO values were 77 and 162 μmol/l, respectively (Table 1; Figure 2). In addition to BWO measurements, pore water oxygen values were determined at six sites (Figure 3). Oxygen microprofiles reveal that oxygenated pore waters were present in sediment depths up to 5 mm.
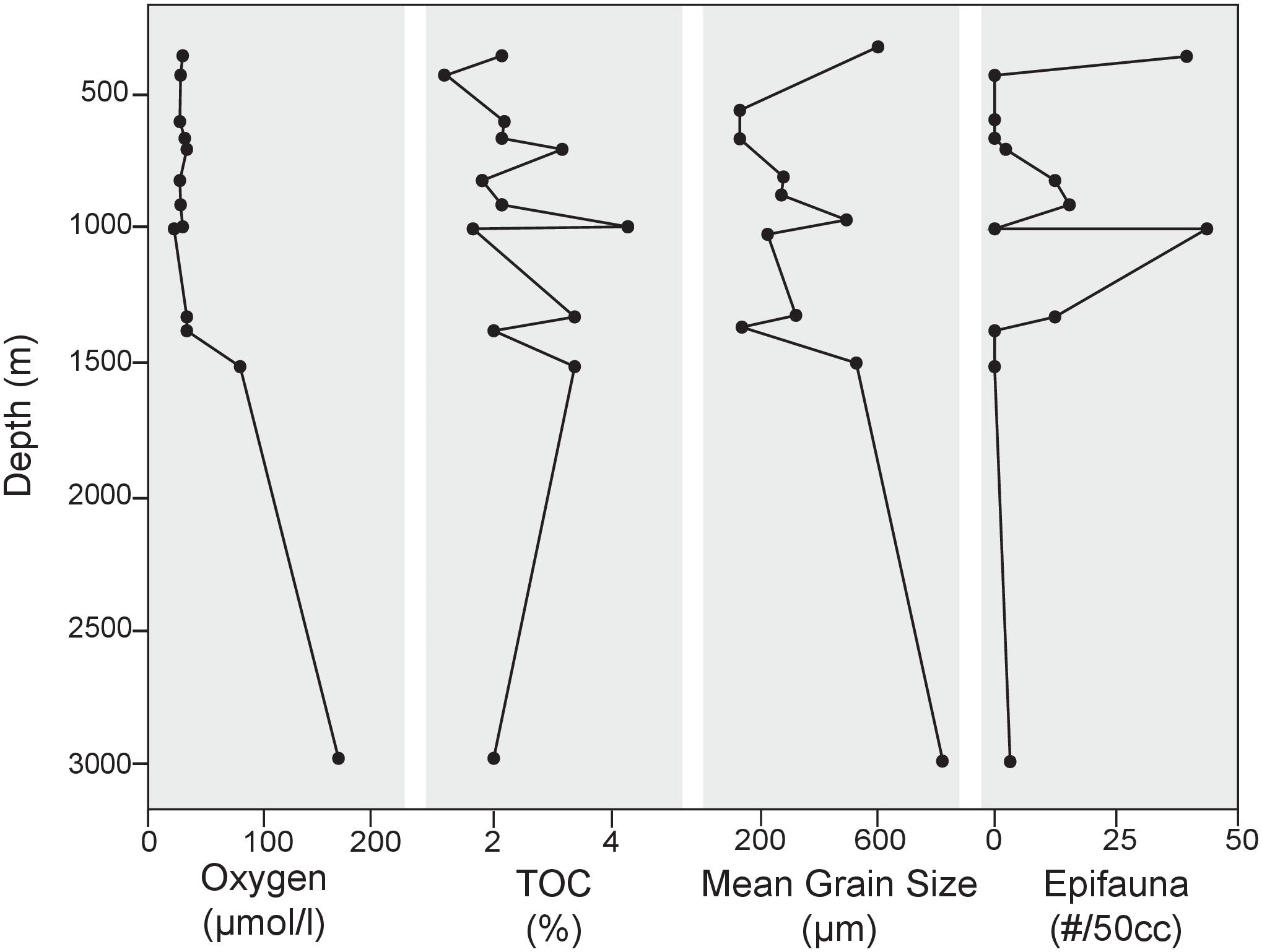
FIGURE 2. Environmental and substrate parameters plotted along the depth transect along with standing stock of epifaunal foraminifera at each site. Mean grain size from particle size analysis lends insight to the heterogeneity of substrates sampled in this study. No apparent relationship between substrate and depth exists in this area.
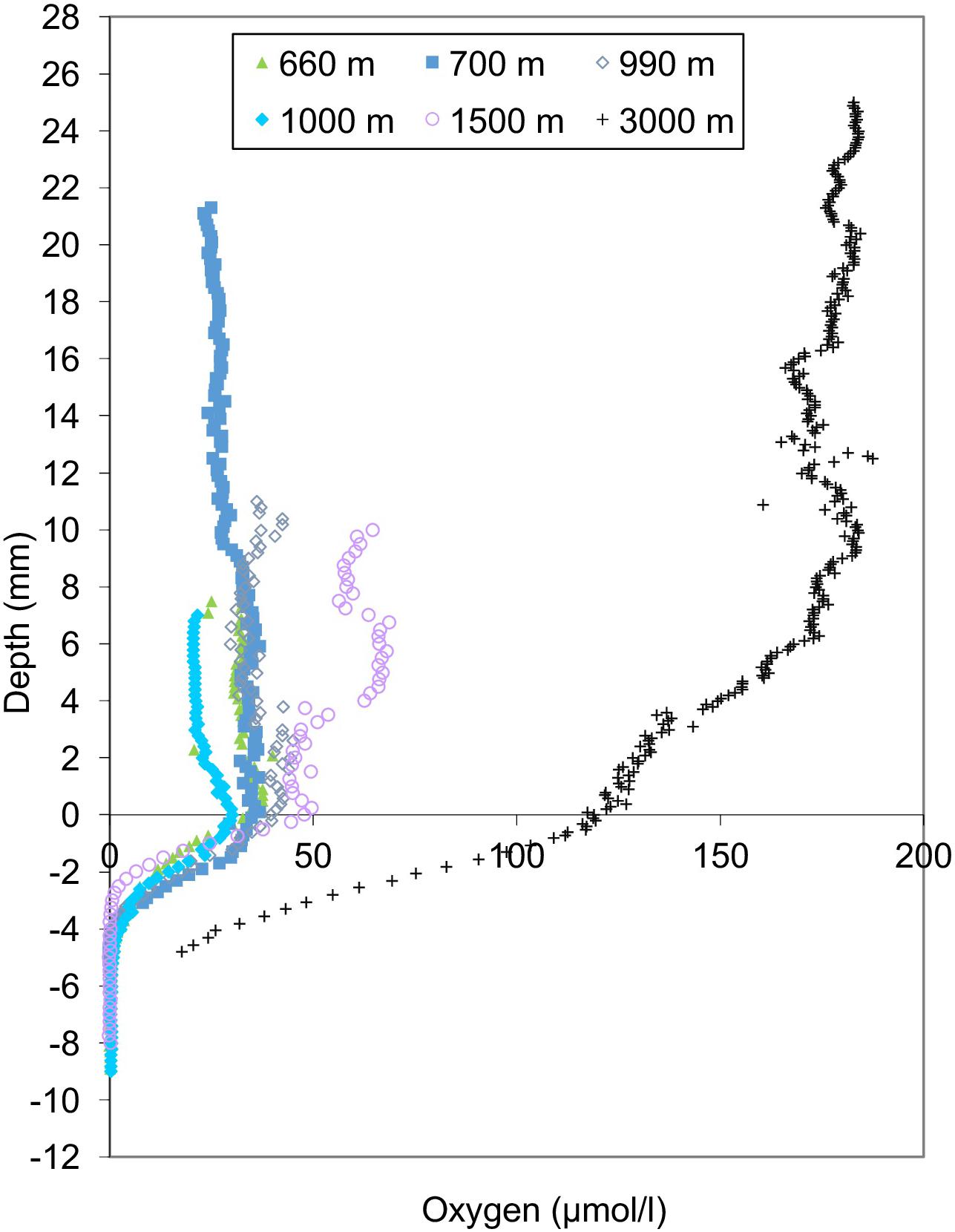
FIGURE 3. Oxygen microprofiles for the six sites observed. Bottom water oxygen measurements from oxygen microprofiles were consistent with CTD casts. Oxygen microprofiles reveal that oxygenated pore waters were present in sediment depths up to 5 mm.
Sediment Properties
Preferential sampling of bathymetric highs and basin edges yielded coarser grained sediments than in basin centers. The cause of the presence of coarse grains in these areas is unknown, but it is likely that bottom currents play a role. Particle size analyses revealed that substrates examined in this study were comprised primarily (41–97% of total sample) of sand-sized, siliciclastic sediments (>63 μm). Appreciable percentages of gravel-sized particles (>2 mm) were also found in several of the samples along the depth transect, namely at 360 (42%) and 2969 m (50.5%). Particle size distribution varied from site to site in this region (Figure 2), with mean grain sizes ranging from 131 μm at 600 m to 829 μm at 2969 m (Figure 2). TOC for the 13 sites along the transect ranged from 1.25 to 4.24% (Table 1; Figure 2 and Supplementary Data Sheet 1).
Surface Sediment Standing Stock
>150 μm
Standing stocks of rose bengal stained specimens from the 0–1 cm interval were determined for all 13 locations (Table 1 and Supplementary Data). On average, surface sediments across the transect contained 164 individuals/50 cm2. Calcareous taxa were more prevalent than agglutinated taxa at the majority of sites across the transect, comprising anywhere from 53 to 98% of the total population. The deepest site on the transect, at 2969 m, had the greatest proportion of agglutinated taxa comprising 47% of the total population.
Maximum abundance of stained foraminifera in the 0–1 cm interval was observed at 700 m (470 individuals/50 cm2). This site was overwhelmingly dominated by Uvigerina peregrina (80% of total population). Uvigerina peregrina was among the most abundant taxon at 10 of the 13 sites across the depth transect, with a maximum at 700 m at 375 individuals/50 cm2. Cassidulina laevigata, Bolivina spissa, Hoeglundina elegans, Uvigerina auberiana, and Cibicidoides wuellerstorfi, were also among the most abundant taxa in the >150 μm size fraction of cores examined in this study (Figure 4).
63–150 μm
Standing stock abundances of the 0–1 cm interval were determined for six locations, 360, 600, 700, 826, 910, and 1000 m to compare the smaller size fraction with the >150 μm fraction. Since foraminiferal populations tend to be smaller in size in organic-rich, oxygen-poor environments (e.g., Perez-Cruz and Machain-Castillo, 1990), examination of this size fraction (despite the time-consuming nature of this effort) provides information for a larger, and more representative percentage of the population. On average, surface sediments across the transect contained 148 individuals/50 cm2 (SD:111). Similar to the >150 μm size fraction, calcareous taxa comprise the majority of the population (87–100%). Stained agglutinated taxa are only present in this size fraction at 826, 910, and 1000 m. Dominant taxa in the 63–150 μm portion were not necessarily the same as those in the >150 μm size fraction. Among the most common taxa of the 63–150 μm fraction were Bolivina spissa, Cassidulina carinata, Buliminella tenuata, Cibicidoides bradyi, Uvigerina proboscidea, and Uvigerina peregrina. Bolivina spissa, the most abundant taxon in the 63–150 μm assemblage, had a peak abundance at 700 m with 128 individuals/50 cm2. While most abundant in the larger size fraction, Uvigerina peregrina was only present at the 910 and 1000 m site in the 63–150 μm size fraction. In order to best understand the community over a range of sizes, the 63–150 μm was combined with the >150 μm portion and will be referred to henceforth as the “> 63 μm population” (Figure 5).
Epifauna
Epifaunal taxa present in cores from the SCB include C. wuellerstorfi and Hanzawaia nipponica. Contrary to expectations based on previous studies (i.e., BFOI-Kaiho, 1994), epifaunal taxa had a marked presence at 12 of the 13 sites analyzed, comprising as much as 35.7% of the total population despite oxygen-poor conditions. The presence and abundance of both taxa do not appear to be associated with changes in any of the measured environmental parameters (i.e., oxygen, TOC, grain size, and temperature; Figure 2). C. wuellerstorfi was present in the >150 μm portion of seven of the 13 sites, comprising 0.28–14.5% of the total population in the surface sediments. H. nipponica was present in abundance at four of the shallowest sites along the transect (360, 429, 600, and 700 m), with maximum abundances at 600 m, dominating the total population at that site and comprising 47% of the total benthic foraminiferal population.
Diversity and Dominance
>150 μm
A total of 60 taxa were identified in this region with 51 calcareous and 9 agglutinated in the >150 μm size fraction. Species richness was variable both across the transect and within different sections of the OMZ (Figure 6). Interestingly, the highest species richness value (27) was found at 1000 m, where BWO values were the lowest. In the dysoxic zone, richness varied from 7 to 23; while the two deepest stations had richness values of 13 and 18. Species were distributed relatively evenly (0.62–0.88), with the exception of the site at 700 m with an evenness value of 0.34.
Shannon diversity (H) of stained foraminifera varied across the transect. Maximum diversity (2.5) was observed at 1378 m. At 700 m depth, with a value of 1.05, we found the lowest diversity value of the sites examined in this study. Despite having the lowest BWO in the study area, the 1000 m had a diversity near the high end of the study area range (2.22). The two deepest sites, had similar diversities (2.25–2.37).
63–150 μm
The >63 μm fraction was analyzed for faunal distribution in the surface sediments at six locations (Figure 7) where 60 calcareous and 10 agglutinated taxa were found in the >63 μm fraction. Species richness was variable, ranging from 10 to 27, with the lowest and highest values being found at 600 and 700 m, respectively. At 1000 m, where oxygen values were the lowest along the transect, richness was among the highest of sites examined for this size fraction (26). Evenness was similar for five of the six sites, with values ranging from 0.70–0.85. Minimum diversity values of 1.62 and 1.82 were found at 600 and 700 m, respectively. Sites exposed to dysoxic BWO had similar trends in richness, standing stock, and BWO. At 1000 m, where BWO values were the lowest of those examined in this study, richness and diversity values were among the highest (26 and 2.5, respectively).
Discussion
Projected increases in size and location of OMZs and other dissolved oxygen-depleted marine habitats associated with climate change highlight the importance of understanding the responses of benthic ecosystems to deoxygenation (e.g., Kamykowski and Zentara, 1990; Sarmiento et al., 1998; Keeling and Garcia, 2002; Helly and Levin, 2004; Stramma et al., 2008; Meier et al., 2011). Benthic environments with limited oxygen availability are typically characterized by high organic carbon contents (high primary productivity), high sediment accumulation rates, fine grain size, low bioturbation, and generally homogeneous habitats (e.g., Reichart et al., 1998; Levin, 2003). As a result of their densities in oxygen-poor environments, and their fossil presence in high-resolution geologic records from OMZs, benthic foraminifera living in oxygen-poor habitats have been the focus of a number of studies (e.g., Sen Gupta and Machain-Castillo, 1993; Bernhard and Alve, 1996; Bernhard and Sen Gupta, 1999; Bernhard et al., 2000). It is well documented that foraminiferal assemblages collected from the soupy, organic-rich sediments of oxygen-poor shelf/slope habitats, including those of the California Borderlands Basins, are overwhelmingly dominated by infaunal taxa (e.g., Bernhard and Reimers, 1991; Silva et al., 1996; Gooday et al., 2009; Gooday and Jorissen, 2012). Enhanced foraminiferal diversity has been reported along OMZ boundaries (e.g., Phleger and Soutar, 1973), and since foraminifera are the predominate fauna persisting through an OMZ, there may be an enhanced regional diversity resulting from boundary effects and habitat heterogeneity (Mullins et al., 1985; Gooday et al., 2009; Gooday et al., 2010). In this study, there do not seem to be any boundary effects that could be related to changes in water properties, including dissolved oxygen. Differences in surficial sediment characteristics of the sampling sites in the present study likely create habitat heterogeneity, enhancing diversity, but infaunal taxa remain dominant in coarser-grained sites as they are in the fine-grained muds typical of organic-rich basins. Results from our study also show that epifauna can comprise an appreciable percentage (up to 35.7%) of assemblages in coarse-grained habitats with dissolved BWO values as low as 23 μmol/l.
Previous studies of living foraminifera in the region primarily sampled fine-grained sediments that typify high productivity environments (e.g., Phleger and Soutar, 1973; Bernhard and Reimers, 1991; Silva et al., 1996). Studies of living foraminifera off California reported that nearby study sites had a complete absence of epifaunal taxa in the Santa Barbara Basin with BWO values of 4.5 μmol/l (Bernhard and Reimers, 1991) and in the San Pedro Basin 2.3–18 μmol/l (Silva et al., 1996). Our results from the SCB show that, in many cases, foraminiferal assemblages collected from the edges of basins and on the ridges between basins have marked differences in assemblages when compared those found in nearby basin-focused studies of Phleger and Soutar (1973), Bernhard and Reimers (1991), and Silva et al. (1996). Basin ridges are characterized by coarser sediments (80–99% of the surface sediments are comprised of sand and gravel sized particles) (>63 μm), probably due to removal of fine-grained sediment by winnowing currents. In the Gulf of Cadiz, appreciable densities of epifauna, including several species of Cibicidoides and Planulina, were present on hard or gravel-laden substrates (Schönfeld, 2002). Schönfeld (2002) noted a relationship between coarse sediments and epifauna in the northeastern Atlantic, and suggested that at least some epifaunal foraminifera were likely to need currents for food acquisition. Schönfeld (2002) proposed that paleo-current velocities could be assessed by examining percentages of epifauna. Although we cannot evaluate the influence of currents on the foraminifera in our study area, at the micron scale, it would not require much energy to create flow around an epifaunal foraminiferan. We suggest the presence of attachment surfaces is the primary influence enhancing epifaunal percentages in our study area. Elevated epibenthic taxa (those that live attached above the average sediment-water interface) prefer a hard substrate for attachment. C. wuellerstorfi is commonly found attached to hard surfaces at or above the sediment-water interface (e.g., Lutze and Thiel, 1989; Burkett et al., 2016). Other unattached epifauna may also prefer access to bottom water than to be surrounded by sediment and pore water. Grain size analyses, in this study, were based on sediment samples from surface materials, and we cannot know how many of the coarse grains were at the sediment-water interface and available for attachment. As a result, a strong correlation between grain size results and all epifaunal taxa may not be expected. Nevertheless, at sites in this study where peak percentages of C. wuellerstorfi were present (>9% of population; 360, 826, 910, and 1000 m), the surface sediments were comprised primarily of sand and gravel-sized particles (95.4–99.7%; Figure 8).
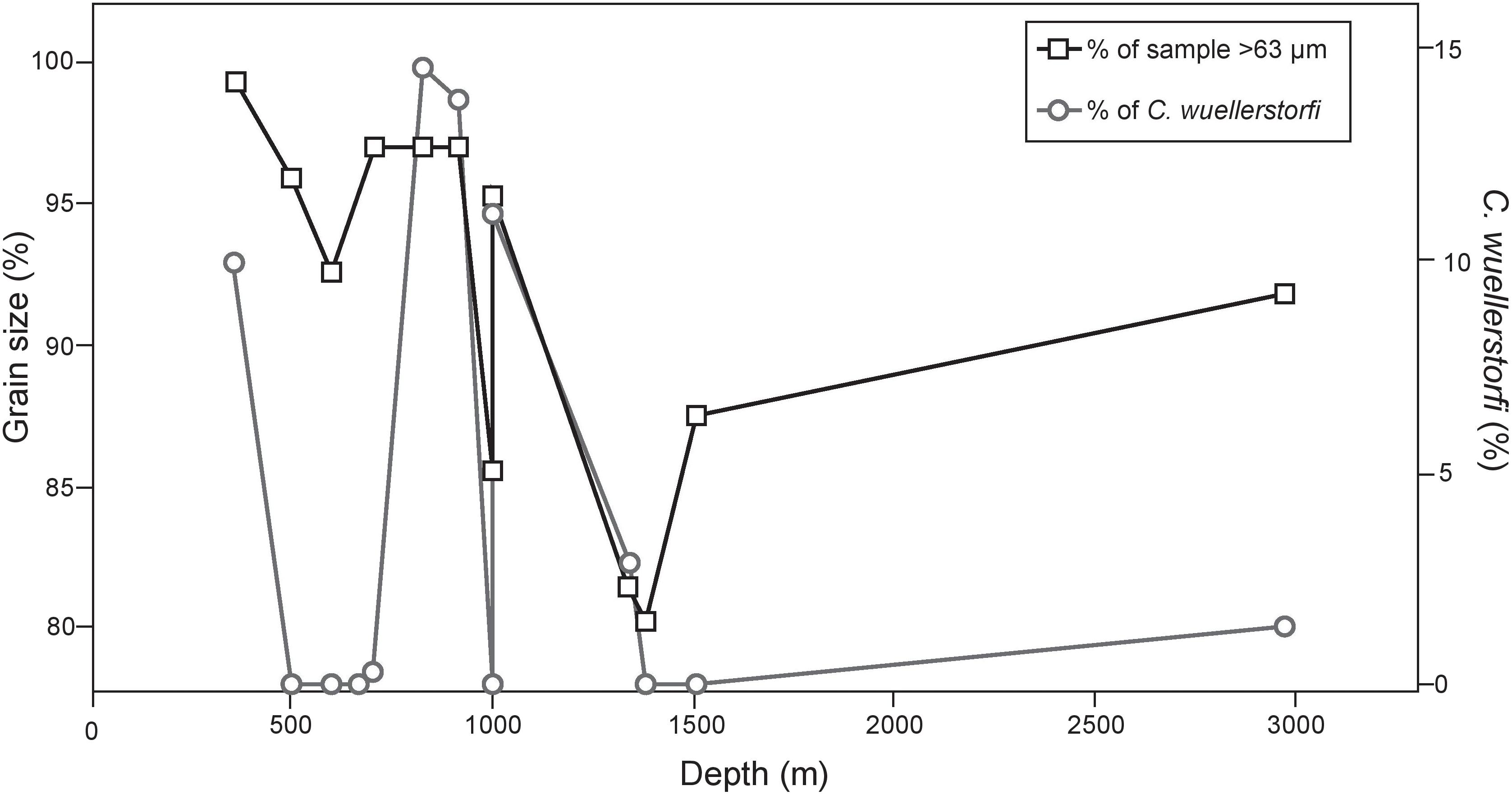
FIGURE 8. Abundance of sand-sized particles or greater characterizing the substrate along with presence of C. wuellerstorfi at each depth. We demonstrate that substrates with greater concentrations of sand-sized particles may provide habitat stability for C. wuellerstorfi to persist, despite low bottom water oxygen concentrations.
Other epifauna (H. nipponica) did not show any apparent relationship with sedimentary characteristics. The sites in which the lowest BWO concentrations and TOC were found had some of the highest concentrations of C. wuellerstorfi. However, BWO and TOC, did not appear to influence the distribution of epifauna as a whole in our study (Figure 2). Typical organic-rich, oxygen-poor seafloor environments are characterized by fine-grained, soupy sediments that have few, if any hard substrates at the sediment-water interface. These environments are dominated by infaunal foraminifera that are adapted for reduced oxygen availability in pore waters (e.g., Bernhard, 1986; Sen Gupta and Machain-Castillo, 1993; Bernhard and Sen Gupta, 1999; Jorissen et al., 2007). Although oxygen and food availability are important limiting variables, we argue that, for at least some epifaunal taxa, the availability of hard substrate is a primary factor that limits their distribution.
Physiological limitations of deep-sea foraminifera have commonly been inferred from specimen distribution patterns collected from available core tops (Kaiho, 1991, 1994, 1999; Jannink et al., 2001; Schmiedl et al., 2003). These inferences have led to commonly held ideas about the oxygen requirements of some foraminiferal taxa. Although the lower limitations of BWO concentrations for epifauna are not yet well understood (Jorissen et al., 2007), several methods use deep-sea foraminiferal species or groups of taxa to assess BWO concentrations. Most of these methods regard epifauna as indicators of well-oxygenated environments. Species of epifaunal Cibicidoides, in particular, are deemed “oxic indicator species” (reviewed in Jorissen et al., 2007).
Despite oxygen-poor conditions in the SCB, epifaunal “oxic indicator” taxa were present at most (12) of the sites observed. Previous studies found C. wuellerstorfi in BWO concentrations as low as 71.5 μmol/l in the SCB (Bernhard and Reimers, 1991), and a recent global study that included specimens from the SCB, found living C. wuellerstorfi in habitats ranging from ∼2 to 277 μmol/l with abundant specimens below 45 μmol/l (Rathburn et al., 2018). A year-long study off the coast of Oregon found that over one-thousand C. wuellerstorfi had colonized hard, artificial substrates in BWO concentrations of ∼11 μmol/l (Burkett et al., 2016). In a colonization study at 4000 m off the coast of California, the epifaunal species Cibicides lobatulus, was the most abundant organism attached to glass rods after a year on the seafloor well below the lysocline (Beaulieu, 2001). Results from our study are consistent with these findings, and support the idea that hard substrates that enable epifaunal species to maintain their position at or above the sediment-water interface are an important factor in the distribution of C. wuellerstorfi, and probably other epifaunal species. Heterogeneity of habitat plays a critical role in the diversity of benthic fauna, including those within oxygen-poor environments, and we suggest that the availability of surfaces for epifaunal attachment influences habitat heterogeneity and the presence of epifaunal foraminifera in oxygen-stressed conditions.
Vertical distribution patterns observed here are consistent with those reported in previous studies of rose bengal stained foraminifera. As expected, C. wuellerstorfi have epifaunal microhabitat preferences with population maxima in the top 1 cm of sediment (Corliss, 1985; Rathburn and Corliss, 1994; Rathburn et al., 1996; Figure 9). H. nipponica also has population maxima in the top 1 cm of sediment in this study, and together with its planoconvex morphology, indicate that this species is epifaunal (Figure 9). Other dominant species, typically classified as infaunal based on their vertical distribution patterns below 1 cm in sediment depth in other regions (U. peregrina and C. laevigata) also show population maxima in the upper 1 cm, with few or no individuals persisting to the lower sections of the sediment column. Hoeglundina elegans had density maxima in the top 1-cm of sediments in this study. It is noteworthy that Hoeglundina elegans is typically found in the top 1-cm of sediments and has been characterized as an epifaunal species by some workers (e.g., Corliss, 1985; Rathburn and Corliss, 1994; Rathburn et al., 1996), but has been considered as an infaunal species by others (e.g., Fontanier et al., 2006). Regardless of the microhabitat preference of H. elegans, it has previously been associated with high oxygen concentrations and relatively low food availability (Schmiedl et al., 1997; Lutze and Coulbourn, 1984; Koho et al., 2008), therefore it is worth mentioning that this species can adapt to a wide range of environmental conditions. The infaunal designation may have resulted from the common association of this species with oxygen-poor habitats. Nevertheless, to be conservative, we refrained from including this species as an epifaunal taxon. In our study, H. elegans was present at all but two sites along the transect, with maximum abundance at site E (1339 m), where it comprised 20% of the total benthic foraminiferal population. Bolivina spissa, however, is also regarded as an infaunal taxon and had a population that persisted lower in the sediment column, with population maxima in the 1–1.5 mm section of our cores.
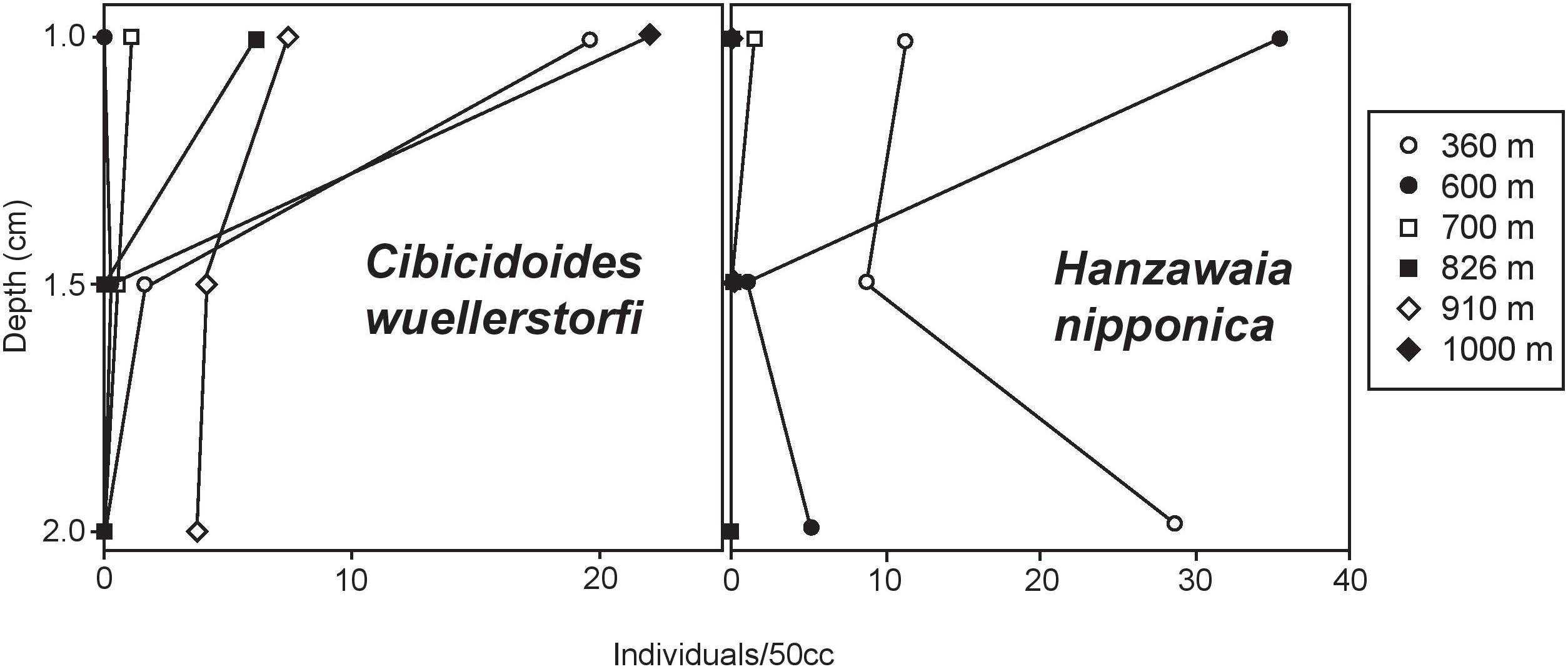
FIGURE 9. Epifaunal foraminiferal verticle distribution within the top 2 cm of sediments analyzed from multicores at six sites along our transect. Epifaunal microhabitat preferences of C. wuellerstorfi are consistent with previous studies. Maximum abundances of H. nipponica in the top cm of sediment were observed at most sites, with the exception of 360 m, suggesting that this taxon can also be described as epifaunal.
As predicted by the TROX model (Jorissen et al., 1995), infaunal taxa dominate assemblages living at the sites examined, comprising 51–100% of the populations in the >150 μm fraction (Figure 4). Thus, despite the heterogeneity of substrates along the transect, infaunal taxa thrive in both the fine- and coarse-grained habitats. Infaunal populations are dominated by Uvigerina peregrina, Cassidulina laevigata, and Bolivina spissa, with varying abundances across the depth transect. Sites with high mean grain size (>500 μm) were dominated by Cassidulina laevigata and Uvigerina peregrina. This differed from the three sites with the lowest mean grain size (<150 μm) which showed an increased presence of less common taxa such as Nonionella stella, Chilostomella oolina, and Globobulimina hoeglundi, which were not observed at other sites with coarser grained substrates. It is possible that in the same way that these coarse grained habitats show an increased abundance of epifaunal taxa, there may be an influence as well on infaunal assemblages.
Results from this study indicate that the epifaunal taxa, C. wuellerstorfi and H. nipponica, can be abundant in habitats where BWO values fall well below the upper threshold of dysoxia. Epifauna such as these are clearly not restricted to well-oxygenated environments, and our results along with those of other recent studies (Burkett et al., 2016; Rathburn et al., 2018), indicate that the physiological limitations of C. wuellerstorfi, and probably other epifauna, should be expanded to include oxygen-poor environments. These results have appreciable consequences for methods that use epifauna for paleo-oxygenation indicators, and provide a greater understanding of the responses of benthic foraminiferal populations to deoxygenation.
Conclusion
Our examination of benthic foraminifera living in coarse-grained sediment environments in the SCB revealed elevated densities of epifaunal taxa in oxygen-poor habitats. In dysoxic conditions (21–28 μmol/l), epifaunal taxa comprised as much as 36% of the living (stained) population at the sites with the coarsest mean grain size. Infaunal assemblages varied between sites with different grain size characteristics, suggesting that substrate may also influence some infaunal taxa. Nevertheless, foraminiferal assemblages in our study were dominated by infaunal taxa, such as Uvigerina and Bolivina, traditionally associated with the supersaturated, unconsolidated mud, characteristic of oxygen-poor habitats, indicating that these taxa are not especially sensitive to substrate type. We suggest that at least some, perhaps many, epifaunal taxa, including C. wuellerstorfi, prefer habitats with coarse grains that allow them to remain at or above the sediment-water interface. It is clear that seafloor habitat heterogeneity influences the distribution of deep-sea benthic foraminifera, including in oxygen-poor environments. These results enhance our ability to predict changes in foraminiferal distribution patterns in deoxygenated seafloor habitats of the future, and also point to the need for consideration of substrate characteristics in assessments of ecological tolerances of foraminiferal species.
Data Availability Statement
The datasets generated for this study can be found in the PANGAEA Data Publisher https://doi.pangaea.de/10.1594/PANGAEA.893323.
Author Contributions
RV processed samples and specimens and performed the analyses. AR, AB, and RV analyzed the data. WZ measured oxygen microprofiles and calibrated California Borderland oxygen measurements. AR and AB collected the samples, and all of the authors contributed to the writing of the paper.
Funding
This material was based in part upon work supported by the National Science Foundation under grant OCE 10-60992 awarded to AR.
Conflict of Interest Statement
The authors declare that the research was conducted in the absence of any commercial or financial relationships that could be construed as a potential conflict of interest.
Acknowledgments
The authors extend sincere gratitude to the captain and crew of the R/V New Horizon and the scientific party aboard NH1108. They also sincerely appreciate help from Jessica Heck for conducting grain size analyses at LacCore (National Lacustrine Core Facility), Department of Earth Sciences, University of Minnesota-Twin Cities.
Supplementary Material
The Supplementary Material for this article can be found online at: https://www.frontiersin.org/articles/10.3389/fmars.2018.00344/full#supplementary-material
References
Altenbach, A., and Sarnthein, M. (1989). “Productivity record in benthic foraminifera,” in Productivity of the Ocean: Present and Past eds W. H. Berger, V.S. Smetacek and G. Wefer (New York: John Wiley), 255–269.
Beaulieu, S. (2001). Life on glass houses: sponge stalk communities in the deep sea. Mar. Biol. 138, 803–817. doi: 10.1007/s002270000500
Bernhard, J. M. (1986). Characteristic assemblages and morphologies of benthic foraminifera from anoxic, organic-rich deposits; Jurassic through Holocene. J. Foramin. Res. 16, 207–215. doi: 10.2113/gsjfr.16.3.207
Bernhard, J. M., and Alve, E. (1996). Survival, ATP pool, and ultrastructural characterization of benthic foraminifera from Drammensfjord (Norway): response to anoxia. Mar. Micropaleontol. 28, 5–17. doi: 10.1016/0377-8398(95)00036-4
Bernhard, J. M., Buck, K. R., Farmer, M. A., and Bowser, S. S. (2000). The santa barbara basin is a symbiosis oasis. Nature 403:77. doi: 10.1038/47476
Bernhard, J. M., Ostermann, D. R., Williams, D. S., and Blanks, J. K. (2006). Comparison of two methods to identify live benthic foraminifera: a test between Rose bengal and celltracker green with implications for stable isotope paleoreconstructions. Paleoceanography 21:PA4210. doi: 10.1029/2006PA001290
Bernhard, J. M., and Reimers, C. E. (1991). Benthic foraminiferal population fluctuations related to anoxia: santa Barbara Basin. Biogeochemistry 15,127–149. doi: 10.1007/BF00003221
Bernhard, J. M., and Sen Gupta, B. K. S. (1999). Foraminifera of Oxygen-Depleted Environments Modern Foraminifera. New York, NY: Springer, 201–216.
BjorkstEdt, E. P., Goericke, R., McClatchie, S., Weber, E., Watson, W., Lo, N., et al. (2011). State of the California Current 2010-2011: regionally variable responses to a strong (but fleeting) La Niña. California Cooper. Oceanic Fish. Invest. Rep. 52, 36–68
Blott, S. J., and Pye, K. (2001). GRADISTAT: a grain size distribution and statistics package for the analysis of unconsolidated sediments. Earth Surf. Process. Landf. 26, 1237–1248. doi: 10.1002/esp.261
Bograd, S. J., and Lynn, R. J. (2003). Long-term variability in the southern California current system. Deep Sea Res. Part II Top. Stud. Oceanogr. 50, 2355–2370. doi: 10.1016/S0967-0645(03)00131-0
Burkett, A. M., Rathburn, A. E., Pérez, M. E., Levin, L. A., and Martin, J. B. (2016). Colonization of over a thousand Cibicidoides wuellerstorfi (foraminifera: schwager, 1866) on artificial substrates in seep and adjacent off-seep locations in dysoxic, deep-sea environments. Deep Sea Res. Part I Oceanogr. Res. Papers 117, 39–50. doi: 10.1016/j.dsr.2016.08.011
Corliss, B. H. (1985). Microhabitats of benthic foraminifera within deep-sea sediments. Nature 314:435. doi: 10.1038/314435a0
Emery, K. O. (1960). The Sea off Southern California, a Modern Habitat of Petroleum. ıOakland, CA: University of California.
Fontanier, C., Mackensen, A., Jorissen, F. J., Anschutz, P., Licari, L., and Griveaud, C. (2006). Stable oxygen and carbon isotopes of live benthic foraminifera from the bay of biscay: microhabitat impact and seasonal variability. Mar. Micropaleontol. 58, 159–183. doi: 10.1016/j.marmicro.2005.09.004
Gooday, A., Levin, L., da Silva, A. A., Bett, B., Cowie, G., Dissard, D., et al. (2009). Faunal responses to oxygen gradients on the Pakistan margin: a comparison of foraminiferans, macrofauna and megafauna. Deep Sea Res. Part II Top. Stud. Oceanogr. 56, 488–502. doi: 10.1016/j.dsr2.2008.10.003
Gooday, A. J., Bett, B. J., Escobar, E., Ingole, B., Levin, L. A., Neira, C., et al. (2010). Habitat heterogeneity and its influence on benthic biodiversity in oxygen minimum zones. Mar. Ecol. 31, 125–147. doi: 10.1111/j.1439-0485.2009.00348.x
Gooday, A. J., and Jorissen, F. J. (2012). Benthic foraminiferal biogeography: controls on global distribution patterns in deep-water settings. Annu. Rev. Mar. Sci. 4, 237–262. doi: 10.1146/annurev-marine-120709-142737
Gorsline, D., Kolpack, R., Karl, H., Drake, D., Thornton, S., Schwalbach, J., et al. (1984). Studies of fine-grained sediment transport processes and products in the California Continental Borderland. Geological Soc. Lond. Special Public. 15, 395–415. doi: 10.1144/GSL.SP.1984.015.01.26
Hammer, Ø., Harper, D., and Ryan, P. (2001). PAST-Palaeontological Statistics. Available at: www.uv.es/~pardomv/pe/2001_1/past/pastprog/past.pdf, acessado em.
Helly, J. J., and Levin, L. A. (2004). Global distribution of naturally occurring marine hypoxia on continental margins. Deep Sea Res. Part I Oceanogr. Res. Papers 51, 1159–1168. doi: 10.1016/j.dsr.2004.03.009
Hendy, I. L., and Kennett, J. P. (2000). Dansgaard-oeschger cycles and the california current system: planktonic foraminiferal response to rapid climate change in Santa Barbara Basin, ocean drilling program hole 893A. Paleoceanography 15, 30–42. doi: 10.1029/1999PA000413
Jannink, N. T., Duijnstee, I., and van der Zwaan, B. (2001). Foraminiferal Patterns in Two Trophically Different Regions: The Northern Adriatic Sea and The Southern Levantine Basin. Available at: http://dspace.library.uu.nl/handle/1874/329017
Jorissen, F. J., de Stigter, H. C., and Widmark, J. G. (1995). A conceptual model explaining benthic foraminiferal microhabitats. Mar. Micropaleontol. 26, 3–15. doi: 10.1016/0377-8398(95)00047-X
Jorissen, F. J., Fontanier, C., and Thomas, E. (2007). Chapter seven paleoceanographical proxies based on deep-sea benthic foraminiferal assemblage characteristics. Dev. Mar. Geology 1, 263–325. doi: 10.1016/S1572-5480(07)01012-3
Kaiho, K. (1991). Global changes of Paleogene aerobic/anaerobic benthic foraminifera and deep-sea circulation. Palaeogeogr. Palaeoclimatol. Palaeoecol. 83, 65–85. doi: 10.1016/0031-0182(91)90076-4
Kaiho, K. (1994). Benthic foraminiferal dissolved-oxygen index and dissolved-oxygen levels in the modern ocean. Geology 22, 719–722. doi: 10.1130/0091-7613(1994)022<0719:BFDOIA>2.3.CO;2
Kaiho, K. (1999). Evolution in the test size of deep-sea benthic foraminifera during the past 120 my. Mar. Micropaleontol. 37, 53–65. doi: 10.1016/S0377-8398(99)00011-0
Kamykowski, D., and Zentara, S. -J. (1990). Hypoxia in the world ocean as recorded in the historical data set. Deep Sea Res. Part A. Oceanogr. Res. Papers 37, 1861–1874. doi: 10.1016/0198-0149(90)90082-7
Karstensen, J., Stramma, L., and Visbeck, M. (2008). Oxygen minimum zones in the eastern tropical Atlantic and Pacific oceans. Prog. Oceanogr. 77, 331–350. doi: 10.1016/j.pocean.2007.05.009
Keeling, R. F., and Garcia, H. E. (2002). The change in oceanic O2 inventory associated with recent global warming. Proc. Natl. Acad. Sci. U.S.A. 99,7848–7853. doi: 10.1073/pnas.122154899
Koho, K., Langezaal, A., Van Lith, Y., Duijnstee, I., and Van der Zwaan, G. (2008). The influence of a simulated diatom bloom on deep-sea benthic foraminifera and the activity of bacteria: a mesocosm study. Deep Sea Res. Part I Oceanogr. Res. Papers 55, 696–719. doi: 10.1016/j.dsr.2008.02.003
Levin, L. (2003). Oxygen minimum zone benthos: adaptation and community response to hypoxia. Oceanogr. Mar. Biol. Annu. Rev. 41, 1–45
Loubere, P., and Fariduddin, M. (1999). Benthic foraminifera and the flux of organic carbon to the seabed Modern foraminifera. New York, NY: Springer, 181–199.
Lutze, G. F., and Coulbourn, W. T. (1984). Recent benthic foraminifera from the continental margin of northwest Africa: community structure and distribution. Mar. Micropaleontol. 8, 361–401. doi: 10.1016/0377-8398(84)90002-1
Lutze, G., and Thiel, H. (1989). Epibenthic foraminifera from elevated microhabitats; Cibicidoides wuellerstorfi and Planulina ariminensis. J. Foraminiferal Res. 19, 153–158. doi: 10.2113/gsjfr.19.2.153
McCorkle, D. C., Keigwin, L. D., Corliss, B. H., and Emerson, S. R. (1990). The influence of microhabitats on the carbon isotopic composition of deep-sea benthic foraminifera. Paleoceanography 5, 161–185. doi: 10.1029/PA005i002p00161
Meier, H. M., Andersson, H. C., Eilola, K., Gustafsson, B. G., Kuznetsov, I., Müller-Karulis, B., et al. (2011). Hypoxia in future climates: a model ensemble study for the Baltic Sea. Geophys. Res. Lett. 38:L24608. doi: 10.1007/s13280-013-0475-6
Mullins, H. T., Thompson, J. B., McDougall, K., and Vercoutere, T. L. (1985). Oxygen-minimum zone edge effects: evidence from the central California coastal upwelling system. Geology 13, 491–494. doi: 10.1130/0091-7613(1985)13<491:OZEEEF>2.0.CO;2
Murray, J. W., and Bowser, S. S. (2000). Mortality, protoplasm decay rate, and reliability of staining techniques to recognize ‘living’foraminifera: a review. J. Foraminiferal Res. 30, 66–70. doi: 10.2113/0300066
Nelson, J. R., Beers, J. R., Eppley, R. W., Jackson, G. A., McCarthy, J. J., and Soutar, A. (1987). A particle flux study in the Santa Monica-San Pedro Basin off Los Angeles: particle flux, primary production, and transmissometer survey. Cont. Shelf. Res. 7, 307–328. doi: 10.1016/0278-4343(87)90071-9
Otto, G. H. (1933). Comparative tests of several methods of sampling heavy mineral concentrates. J. Sediment. Res. 3, 30–39. doi: 10.1306/D4268E3C-2B26-11D7-8648000102C1865D
Perez-Cruz, L. L., and Machain-Castillo, M. L. (1990). Benthic foraminifera of the oxygen minimum zone, continental shelf of the Gulf of Tehuantepec, Mexico. J. Foraminiferal Res. 20, 312–325. doi: 10.2113/gsjfr.20.4.312
Phleger, F. B., and Soutar, A. (1973). Production of benthic foraminifera in three east Pacific oxygen minima. Micropaleontology 19, 110–115. doi: 10.2307/1484973
Rathburn, A. E., and Corliss, B. H. (1994). The ecology of living (stained) deep-sea benthic foraminifera from the Sulu Sea. Paleoceanography 9, 87–150. doi: 10.1029/93PA02327
Rathburn, A. E., Corliss, B. H., Tappa, K. D., and Lohmann, K. C. (1996). Comparisons of the ecology and stable isotopic compositions of living (stained) benthic foraminifera from the Sulu and South China Seas. Deep Sea Res. Part I Oceanogr. Res. Pap. 43, 1617–1646. doi: 10.1016/S0967-0637(96)00071-4
Rathburn, A.E., Willingham, J., Ziebis, W., Burkett, A. M., Corliss, B. H. (2018). A New biological proxy for deep-sea paleo-oxygen: pores of epifaunal benthic foraminifera. Sci. Rep. 8:9456 doi: 10.1038/s41598-018-27793-4
Reichart, G. -J., Lourens, L., and Zachariasse, W. (1998). Temporal variability in the northern arabian sea oxygen minimum zone (OMZ) during the last 225,000 years. Paleoceanography 13, 607–621. doi: 10.1029/98PA02203
Revsbech, N. P., Christensen, P. B., Nielsen, L. P., and Sørensen, J. (1989). Denitrification in a trickling filter biofilm studied by a microsensor for oxygen and nitrous oxide. Water Res. 23, 867–871. doi: 10.1016/0043-1354(89)90011-0
Revsbech, N. P., and Jørgensen, B. B. (1986). “Microelectrodes: their use in microbial ecology,” in Advances in Microbial Ecology. K. C. Marshall (New York, NY: Springer), 293–352.
Sarmiento, J. L., Hughes, T. M., Stouffer, R. J., and Manabe, S. (1998). Simulated response of the ocean carbon cycle to anthropogenic climate warming. Nature 393:245. doi: 10.1038/30455
Schmiedl, G., Mackensen, A., and Müller, P. J. (1997). Recent benthic foraminifera from the eastern South Atlantic Ocean: dependence on food supply and water masses. Mar. Micropaleontol. 32, 249–287. doi: 10.1016/S0377-8398(97)00023-6
Schmiedl, G., Mitschele, A., Beck, S., Emeis, K. -C., Hemleben, C., Schulz, H., et al. (2003). Benthic foraminiferal record of ecosystem variability in the eastern Mediterranean Sea during times of sapropel S 5 and S 6 deposition. Palaeogeogr. Palaeoclimatol. Palaeoecol. 190, 139–164. doi: 10.1016/S0031-0182(02)00603-X
Schönfeld, J. (2002). A new benthic foraminiferal proxy for near-bottom current velocities in the Gulf of Cadiz, northeastern Atlantic Ocean. Deep Sea Res. Part I Oceanogr. Res. Papers 49, 1853–1875. doi: 10.1016/S0967-0637(02)00088-2
Sen Gupta, B. K. S., and Machain-Castillo, M. L. (1993). Benthic foraminifera in oxygen-poor habitats. Mar. Micropaleontol. 20, 183–201. doi: 10.1016/0377-8398(93)90032-S
Silva, K. A., Corliss, B. H., Rathburn, A. E., and Thunell, R. C. (1996). Seasonality of living benthic foraminifera from the san pedro basin, California Borderland. Oceanogr. Literat. Rev. 9:916.
Stott, L.D., Hayden, T.P., and Griffith, J. (1996). Benthic foraminifera at the Los Angeles county whites point outfall revisited. J. Foraminiferal Res. 26, 357–368. doi: 10.2113/gsjfr.26.4.357
Keywords: benthic foraminifera, epifauna, infauna, oxygen, Southern California Bight
Citation: Venturelli RA, Rathburn AE, Burkett AM and Ziebis W (2018) Epifaunal Foraminifera in an Infaunal World: Insights Into the Influence of Heterogeneity on the Benthic Ecology of Oxygen-Poor, Deep-Sea Habitats. Front. Mar. Sci. 5:344. doi: 10.3389/fmars.2018.00344
Received: 27 May 2018; Accepted: 06 September 2018;
Published: 17 October 2018.
Edited by:
Arthur Capet, MAST – University of Liège, BelgiumReviewed by:
Gerhard Schmiedl, Universität Hamburg, GermanyUrsula Felicitas Marianne Witte, University of Aberdeen, United Kingdom
Copyright © 2018 Venturelli, Rathburn, Burkett and Ziebis. This is an open-access article distributed under the terms of the Creative Commons Attribution License (CC BY). The use, distribution or reproduction in other forums is permitted, provided the original author(s) and the copyright owner(s) are credited and that the original publication in this journal is cited, in accordance with accepted academic practice. No use, distribution or reproduction is permitted which does not comply with these terms.
*Correspondence: Ryan A. Venturelli, raventurelli@mail.usf.edu
†Present address: Ryan A. Venturelli, College of Marine Science, University of South Florida, St. Petersburg, FL, United States; Anthony E. Rathburn, Department of Geological Sciences, California State University, Bakersfield, CA, United States; Ashley M. Burkett, Boone Pickens School of Geology, Oklahoma State University, Stillwater, OK, United States