Integrating Diel Vertical Migrations of Bioluminescent Deep Scattering Layers Into Monitoring Programs
- 1OceanLab, Department of Physics and Earth Sciences, Jacobs University, Bremen, Germany
- 2Functioning and Vulnerability of Marine Ecosystems Group, Department of Renewable Marine Resources, Instituto de Ciencias del Mar (ICM-CSIC), Barcelona, Spain
- 3Institut de Recherche pour le Développement (IRD), Centre National de la Recherche Scientifique (CNRS), Aix Marseille Université, Université de Toulon, Marseille, France
- 4SARTI, Universitat Politècnica de Catalunya (UPC), Barcelona, Spain
- 5Laboratori Nazionali del Sud LNS, Istituto Nazionale di Fisica Nucleare (INFN), Frascati, Italy
- 6Stazione Zoologica Anton Dohrn (SZN), Naples, Italy
- 7Dipartimento di Scienze della Vita e dell’Ambiente, Università Politecnica delle Marche (UNIVPM), Ancona, Italy
- 8Ocean Networks Canada (ONC), University of Victoria, Victoria, BC, Canada
- 9Department of Biology, University of Victoria, Victoria, BC, Canada
The deep sea (i.e., >200 m depth) is a highly dynamic environment where benthic ecosystems are functionally and ecologically connected with the overlying water column and the surface. In the aphotic deep sea, organisms rely on external signals to synchronize their biological clocks. Apart from responding to cyclic hydrodynamic patterns and periodic fluctuations of variables such as temperature, salinity, phytopigments, and oxygen concentration, the arrival of migrators at depth on a 24-h basis (described as Diel Vertical Migrations; DVMs), and from well-lit surface and shallower waters, could represent a major response to a solar-based synchronization between the photic and aphotic realms. In addition to triggering the rhythmic behavioral responses of benthic species, DVMs supply food to deep seafloor communities through the active downward transport of carbon and nutrients. Bioluminescent species of the migrating deep scattering layers play a not yet quantified (but likely important) role in the benthopelagic coupling, raising the need to integrate the efficient detection and quantification of bioluminescence into large-scale monitoring programs. Here, we provide evidence in support of the benefits for quantifying and continuously monitoring bioluminescence in the deep sea. In particular, we recommend the integration of bioluminescence studies into long-term monitoring programs facilitated by deep-sea neutrino telescopes, which offer photon counting capability. Their Photo-Multiplier Tubes and other advanced optical sensors installed in neutrino telescope infrastructures can boost the study of bioluminescent DVMs in concert with acoustic backscatter and video imagery from ultra-low-light cameras. Such integration will enhance our ability to monitor proxies for the mass and energy transfer from the upper ocean into the deep-sea Benthic Boundary Layer (BBL), a key feature of the ocean biological pump and crucial for monitoring the effects of climate-change. In addition, it will allow for investigating the role of deep scattering DVMs in the behavioral responses, abundance and structure of deep-sea benthic communities. The proposed approach may represent a new frontier for the study and discovery of new, taxon-specific bioluminescence capabilities. It will thus help to expand our knowledge of poorly described deep-sea biodiversity inventories and further elucidate the connectivity between pelagic and benthic compartments in the deep-sea.
Introduction
The deep sea (i.e., >200 m depth) is the largest biome of the planet. It represents the 65% of the whole planet’s surface and contains more than 95% of the biosphere, with more than three quarters of the ocean’s surface projecting to depths below 3,000 m (Costello et al., 2010; Haddock et al., 2017; Sweetman et al., 2017). Unfortunately, only a minimal percentage of the deep sea has been explored in terms of its biological components, and therefore most of marine biodiversity remains uncensused (Mora et al., 2011; Snelgrove, 2016). Species accumulation curves obtained from a range of deep-sea studies to date do not reach an asymptotic plateau, indicating that the cataloged number of species does not come close to the true species richness (Danovaro et al., 2010; Webb et al., 2010; Costello et al., 2012). In fact, on Earth, of all classified species only 16% are marine (Costello and Chaudhary, 2017). During the second half of the previous century, the prevalent perception of an isolated benthic environment, with relative stability in terms of hydrodynamism and associated oceanographic conditions, was overturned (Hessler and Sanders, 1967; Levin et al., 2010; Ramirez-Llodra et al., 2010; Levin and Sibuet, 2012; Smith et al., 2017). Additionally, it was considered oligotrophic and sustaining low biomass and biodiversity, which in turn was causing underestimations of global species abundance and biomass (reviewed in Rex et al., 2006; Thurber et al., 2014).
Nowadays this perception has changed drastically and, although population densities are low and clustered, and while depth-related decreasing trends exist globally, this biome musters higher than expected biodiversity (Danovaro et al., 2010; Costello and Chaudhary, 2017) and carbon turnover rates through biological mechanisms (Snelgrove et al., 2017). Moreover, new knowledge has been gathered on the tight linkages between benthic ecosystems in continental margins or abyssal oceanic plains and the pelagic zone above. Such linkages are either expressed as the settling of food falls and pulses of organic matter (Davies et al., 2006; Aguzzi et al., 2012b, 2018; Thomsen et al., 2017), resuspension due to wind-driven upwelling (Allen and Durrieu de Madron, 2009), or are actively mediated by animal behavior, with vertical displacements taking place throughout the water column (Steinberg et al., 2008; Schmidt et al., 2011; Drazen and Sutton, 2017; Griffiths et al., 2017). These movements, when occurring on a diel (i.e., 24-h) basis, are known as Diel Vertical Migrations (DVMs; Brierley, 2014). DVMs are primarily driven by trade-off strategies balancing the elevated risk of visual predation vs. the benefit of using light in the search for prey, as animals utilize background light for their feeding strategies (Hays et al., 2010). A depth- and light-related niche partitioning may generate a series of synchronized vertical movements of predators and preys within the adjacent depth strata (i.e., staged migrations) or those movements could even occur in a single run (Naylor, 2005; Aguzzi and Company, 2010; Brierley, 2014). Along continental margins on the middle and lower slopes, as well as at abyssal depths, animals could approach or even enter the ecotone between the water column and the benthic ecosystems (i.e., the benthic boundary layer; BBL) at a certain time of the day, acting as predators or prey, hence being vectors of carbon and energy.
One important agent for the transfer of carbon and energy between benthic and pelagic ecosystems is the formation of deep scattering layers, i.e., aggregations of invertebrates and vertebrates driven by ecological needs, including food acquisition, reproduction or avoiding predators (Dietz, 1962). Since physical and chemical gradients in the ocean are generally stronger in the vertical rather than in the horizontal axis over comparable spatial scales, they result in these horizontal large layers of organisms (Benoit-Bird et al., 2017; Sato and Benoit-Bird, 2017). Through the exchange of energy across adjacent oceanic layers at rates faster than the ones dictated by passive sinking and hydrodynamically-induced vertical mixing, the migrating animals enhance the efficiency of the biological pump, sustain food webs, and provide temporal triggers for deep-sea communities, ultimately contributing to the vertical connectivity in the marine environment (Bianchi et al., 2013; Davison et al., 2013; Ochoa et al., 2013; Irigoien et al., 2014; Kelly et al., 2019). As such, for the majority of benthic ecosystems (i.e., apart from the occasional spots of chemosynthetic primary productivity; e.g., Tunnicliffe et al., 2003), benthopelagic coupling remains the principal—if not sole—path that provides the energy to sustain their functions, as well as the structure and biomass of their communities. A re-evaluation of the mechanism of the biological pump is therefore required, with carbon transfer models including passive sinking, diffusion and advection of dissolved organic matter, alongside the active transport by the vertical migration of animals (Vereshchaka et al., 2019).
A major part of marine organisms produce and emit their own light (a process named bioluminescence). In the water column, more than 75% of all organisms larger than 1 cm, from the surface down to 4,000 m depth, are known from the literature to be bioluminescent (Martini and Haddock, 2017). At the seafloor on the other hand, benthic bioluminescent organisms include between 30 and 40% of all animal taxa (Johnsen et al., 2012; Martini et al., 2019). Bioluminescence is an ecological trait with an important role in relationships between organisms, as it impacts their efficiency of resource acquisition, reproduction, as well as survival (Haddock et al., 2010; Martini et al., 2020a). Since light emission is an ubiquitous functional trait in the ocean, recording and quantifying it in situ has been used as a bio-optical measurement to describe the fine-scale distribution of secondary producers such as dinoflagellates, copepods, euphausiids or gelatinous zooplankton (Nealson et al., 1986; Widder et al., 1999; Cronin et al., 2016; Messié et al., 2019), especially in the less observed zones of the ocean such as mesopelagic depths (St. John et al., 2016).
At meso- and disphotic depths (from 200 to 1,000 m), small-sized mesopelagic fishes, gelatinous zooplankton and crustaceans dominate the deep scattering layer, with the exact taxonomic composition of the migrating layers, however, yet to be determined in most oceanic areas (Kaltenberg et al., 2007; Irigoien et al., 2014; Gjøsæter et al., 2017; Proud et al., 2017; Seki and Polovina, 2019). Industrial fisheries at near-global scales are expected to target the mesopelagic deep scattering layers in years to come, as an exploitable source for aquaculture (e.g., fish and crustaceans’ meal), nutritional supplements and pharmaceutical products (Hidalgo and Browman, 2019; Wright et al., 2020). Apart from the impact of this direct pressure, climate-driven changes in oceanographic conditions (Levin and Le Bris, 2015), as well as extreme physico-chemical conditions and energy pollution associated with deep-sea mining (e.g., turbidity and toxic metals in the form of sediment plumes that are discharged during mining activities and the noise generated by operations; Gillard et al., 2019; Drazen et al., 2020; Smith et al., 2020), are also expected to harm the mesopelagic communities associated with the areas where mining might potentially take place, with the potential additive effects on resident communities and their environments still unpredicted to date.
The intensive exploitation of a more or less pristine system such as the twilight zone (Martin et al., 2020), is bound to have repercussions on the active, vertical transfer of carbon and nutrients to the deep seafloor-benthic areas of the planet by DVMs, through the alteration of the complex trophic relationships which can extend down to the BBL (Longhurst and Harrison, 1988; Davison et al., 2013; Klevjer et al., 2016; Aumont et al., 2018). Moreover, a potential weakening of the synchronization that this displacement exerts on the behavioral activity of predators and preys in the deeper benthic realms, both in terms of the onset, offset and total duration of activity phases, should be evaluated in relation to the overall ecosystem functioning (Ochoa et al., 2013; Aguzzi et al., 2015). Indeed, the rhythmic behavior (see section “Biological Rhythms in the Deep Sea” below) of the species constituting any community over the diel and the seasonal basis strongly affects what we perceive as local richness, and therefore our understanding of the food web structure (Bahamon et al., 2009; Hart et al., 2010; Naylor, 2010; Sardà and Aguzzi, 2012; Mat, 2019), an estimation which is mostly based on temporally scattered (non-continuous or of inadequate frequency and/or duration) sampling and monitoring routines (e.g., cruise-based surveys).
Here, we reviewed the available literature to provide the state of the art of deep-sea bioluminescence and propose the integration of its measurements into a strategy for the continuous, long-term in situ monitoring of DVMs. In doing so, the role of bioluminescent species as agents of temporal variability of the depth of the deep scattering layers (which in turn, by interacting with deep benthos, could synchronize the latters’ behavioral rhythms worldwide) can be better understood. As the carbon interchange between the water column and the seabed is an ecosystem function which should be measured at temporal frequencies corresponding to the DVMs and the temporal responses of benthic species within and above the BBL, we provided a conceptual overview of technologies and protocols for the monitoring of bioluminescence. In doing so, we focused on neutrino telescope assets as promising, temporally intensive monitoring sites, increasing their societal value through potential contributions toward fishery management, and merging the interest of two very broad communities: marine and astrophysical scientists.
The Deep-Sea Ecosystems: a Symphony of Cycles and Rhythms
Environmental Cycles and Episodic Signals
Deep-sea hydrodynamic flows are modulated by periodic (e.g., tides) and episodic (e.g., atmospheric patterns) events that drive surface circulation. Surface tides supply much of the mechanical energy required to generate internal tides, as they move stratified water up and down mid-ocean ridges and seamounts, thus producing waves in the ocean’s interior. Internal waves, produced at a tidal frequency, are primary drivers of deep-sea mixing processes (Vic et al., 2019) that modulate the behavior of deep-sea organisms (Aguzzi et al., 2010; Cuvelier et al., 2017).
Surface-generated mesoscale eddies, i.e., circular, ∼100 km wide currents, may be responsible for the creation of deep-sea inertial currents, leading to the transfer of energy from mesoscale to small-scale motions. In the China Sea for example, surface mesoscale eddies have been found to be related to deep-sea current velocities of 0.1 m s–1, with periods of 1–2 months and a 10-fold increase in kinetic energy (i.e., the energy of water due to its motion) (Zhang et al., 2015). In the Eastern Mediterranean Sea, deep-sea cyclonic and anticyclonic events of shorter period (i.e., from quasi inertial to between 5 and 11 days; Rubino et al., 2012; Meccia et al., 2015), derived from bathymetric constraints of abyssal circulation patterns, have been detected, showing energetic episodes with current intensities that may reach up to 0.15 m s–1, effectively contributing to deep-sea mixing processes (Meccia et al., 2015).
Episodic events such as benthic storms, increasing bottom-water turbidity in the deep ocean, are primarily created by deep cyclones and can take place at different temporal and spatial scales. These storms may last from a few hours to a few weeks, covering distances from several hundred meters to several hundred kilometers, when related to internal, slow-moving Rossby waves (Gross and Williams, 1991) produced by the effect of Earth’s rotation on ocean circulation. The storms, generally linked to current speeds exceeding 0.2 m s–1 (Hollister and McCave, 1984), are able to move and resuspend vast quantities of sediments from the seabed, leading to the formation of benthic nepheloid (turbid) layers (Gross and Williams, 1991; Gardner et al., 2017). These nepheloid layers are absent or weak in deep-sea areas subject to relatively low eddy kinetic energy events (Gardner et al., 2017). Benthic storms have been detected in areas with high sea-surface eddy kinetic energy, frequently occurring beneath the meandering, e.g., the Argentine Basin (S Atlantic) and the Gulf Stream (N Atlantic) with its associated rings. There, they generate deep cyclones, anticyclones, and topographic waves that in turn create currents with sufficient bed-shear stress to erode and resuspend sediment, thus initiating or enhancing benthic storms (Gardner et al., 2017). Volcanic eruptions and earthquakes can also generate submarine slides and turbidity currents (Aguzzi et al., 2012a; Gardner et al., 2017). The effect of the storms on the benthic environment depends on the stress for deposition and erosion of fine sediments, in turn related to both large-scale topographic effects (100 km) and small-scale bottom roughness (1 cm) caused by benthic infauna (Gross and Williams, 1991).
Winter convective mixing may produce semi-periodic deep-sea storms. In the western Mediterranean, the cooling of surface water in winter eventually increases water density enough to cause cascading down canyons, the continental slope (Canals et al., 2006; Palanques et al., 2009; Puig et al., 2013a), or even in the open sea (Houpert et al., 2016), transporting large amounts of sediment down to depths of 2,400 m, and creating nepheloid layers as thick as 1,500 m. The convection currents may reach speeds >0.6 m s–1 (Canals et al., 2006), exporting large amounts of organic matter toward the sea bottom, unlike the relatively smooth flux of organic matter taking place during winter and spring seasons in years of shallower and discontinuous convective mixing (Bernardello et al., 2012). Similar cooling and cascading phenomena have been measured at high latitudes in the NE and NW Atlantic (Koeve et al., 2002; Puig et al., 2013b). In the subtropics off southern Taiwan (W Pacific), torrential rainfall may also create sufficiently high sediment concentrations to generate turbid hyperpycnal down-canyon flows (Kao et al., 2010).
Finally, moving onto multiannual scales, an increasing frequency of extreme winter conditions linked to climate change may lead toward more often deep-sea convection events (Schroeder et al., 2016), while the spatiotemporal shifting of semi-periodic climate change indicators (Zhang et al., 2011; Srivastava et al., 2020) such as El Niño and the Southern Oscillation (ENSO), the Pacific-North American teleconnection pattern (PNA), the North Atlantic Oscillation (NAO) and the Mediterranean Oscillation (MO), may also alter the known patterns of episodic deep-sea events and the fluxes of sediments and organic matter.
Biological Rhythms in the Deep Sea
Behavioral rhythms of benthic fauna are regularly evident in the form of depth-related, vertical (benthopelagic) or horizontal (nektobenthic) migrations, or stationary emergence/retraction (endobenthic) patterns from/into the seabed (Aguzzi and Company, 2010; Aguzzi et al., 2015; Benoit-Bird et al., 2017). For example, the burrowing habits of the deep-sea Norway lobster (Nephops norvegicus) drive massive population emergence peaks, phased at an optimum light intensity threshold (Chiesa et al., 2010; Sardà and Aguzzi, 2012). At disphotic depths (i.e., >400 m), animals receive different temporal cues substituting sunlight, and utilize them in order to time these populational movements through a synchronization of their biological clocks. For crustacean decapods and fishes, this syncing can either be a result of direct environmental signals such as periodic hydrodynamism (i.e., internal tides and inertial currents; Wagner et al., 2007; Aguzzi et al., 2010; Doya et al., 2014), changes in water temperature and salinity (Matabos et al., 2014) and phytopigment and oxygen concentrations (Chatzievangelou et al., 2016), or can be indirectly induced by the intermittent presence of massive numbers of predators and prey from a vertically migrating deep scattering layer, which rhythmically come in contact with the BBL (Ochoa et al., 2013; Aguzzi et al., 2018). In the case of the latter, this behaviorally-sustained benthopelagic coupling is to date poorly studied, due to the lack of a sufficient volume of continuous, long-term and high frequency time-series at reference locations in the deep sea. For example, Vereshchaka et al. (2019) reported that vertical migrations are nearly absent from the lower bathypelagic Atlantic zone due to a sharp decrease in the concentration of planktonic food. Aguzzi et al. (2017), however, reported the presence of bioluminescent migrating deep-scattering layers at depths >3 km in the oligotrophic Central Mediterranean (see also section “Monitoring Diel Biological Rhythms in Along the Continental Margin and at Abyssal Areas” below).
Moving toward lower geophysical frequencies, lunar tidal cues (i.e., alternation of spring and neap tide cycles; Talley et al., 2011) are indirectly transferred to the deep sea, with tidally controlled particle fluxes and current regimes (Mercier et al., 2011). Marine organisms adapt to these lunar and semi-lunar cycles mainly by synchronizing their spawning and general reproductive activity (Tessmar-Raible et al., 2011). On the other hand, seasonality may occur with internal time-keeping mechanisms (Helm et al., 2013), calibrated by variations in multiple factors such as environmental variables, the availability of food and energy transfer (e.g., seasonal variations in food falls of primary productivity such as the settling of spring and summer blooms, as well as rapid transfer of detritus in winter) and predator-prey dynamics, or by ontogenetic cycles related to growth and reproduction (Sardà et al., 1994; McClain and Barry, 2010; Lambert et al.,2017a,b; Thomsen et al., 2017; Chauvet et al., 2018). Accordingly, the strength of the rhythmic movements of the deep scattering layer can also follow a seasonal pattern, due to the tuning of reproduction and growth upon photoperiodic (i.e., day-length) changes in photic and disphotic areas, as well as upon variations in carbon-inputs by primary productivity in the deep-sea (Gage and Tyler, 1991). Finally, the intensity of those effects on animal activity and behavior can be latitude-dependent, following the respective clines of tidal phases and solar photoperiod, thus highlighting the multifaceted nature of biological rhythms and the fundamental role of habitat as they were shaped throughout the evolutionary process (Gerkema et al., 2013; Helm et al., 2013; Hut et al., 2013).
Monitoring Diel Biological Rhythms Along the Continental Margin and at Abyssal Areas
Background
Early artisanal fisheries in areas of narrow continental slope were our first point of observational access to the deep-sea fauna (Gordon et al., 2003), providing the first indications of the existence of deep-sea rhythms and resulting in a day/night fishing schedule. Following these empirical observations, scientific tools to obtain field results consisted of classical technologies such as direct sampling by trawling, and were then gradually replaced by more advanced (but still vessel-assisted) imaging tools like towed cameras (Bicknell et al., 2016; Clark et al., 2016) and short-term deployments of camera modules by submersibles (e.g., up to 4 weeks; Tunnicliffe et al., 1990). Indicatively, Aguzzi et al. (2015) depicted benthopelagic coupling with temporally scheduled trawling on the western Mediterranean slope (∼400 m), where synchronized day-night rhythms were detected between benthopelagic and benthic predators and preys. Previously, analysis of melatonin extracted from hauled demersal fishes showed the occurrence of tidally-modulated rhythms (Wagner et al., 2007), while towed surveys showed varying patterns in the activity of two crustacean species (Trenkel et al., 2007). The aforementioned results apply over many crustacean decapods across deep continental margins on a global scale (see Aguzzi and Company, 2010; Aguzzi et al., 2011), and are also corroborated by laboratory experiments, where crustacean locomotion was assessed under conditions of constant darkness and varying hydrodynamic flows (Aguzzi et al., 2007, 2009; Aguzzi and Sardà, 2008; Sbragaglia et al., 2015, 2017; Nuñez et al., 2016).
As more advanced technologies emerged, cabled observatories and landers were used to increase the potential for either higher frequency and/or longer duration for in situ monitoring of activity rhythms in the deep sea. The fauna of a hydrocarbon seep in Sagami Bay, Japan (∼1,100 m) displayed tidally-controlled rhythmicity, with animal responses varying in orders of magnitude (Aguzzi et al., 2010) based on footage of a permanent observatory. At shallower depths in the same area (∼500 m), continuous monitoring of a whale carcass with landers revealed the presence of mostly day-night and occasionally tidal-based rhythms for the majority of the benthic species during the early succession stages (Aguzzi et al., 2018). Various fixed and mobile platforms (i.e., benthic crawler) of the NEPTUNE Cabled Observatory operated by Ocean Networks Canada1 have been used for similar studies, with faunal behavior in a range of aphotic depths being connected to the local tidal regimes and to periodic fluctuations of oceanographic and atmospheric conditions (e.g., Doya et al., 2014; Matabos et al., 2014; Chatzievangelou et al., 2016; Lelièvre et al., 2017). Day-night and tidal-related rhythms have been recently found at the Lofoten-Vesterålen (LoVe) deep-sea observatory in Norway for sessile and motile megafauna such as the bubblegum coral (Paragorgia arborea; Zuazo et al., 2020), a deep, cold-water coral (Lophelia pertusa; Osterloff et al., 2019), shrimps (Osterloff et al., 2016), rockfish (Sebastes sp.; described in Aguzzi et al., 2020b but not formally analyzed yet by chronobiological statistics), and other fauna (Purser, 2015). Moreover, a cabled monitoring module (i.e., TempoMini) evidenced inertial and tidal rhythms in communities of deep hydrothermal vents in both the NE Pacific and Mid-Atlantic (Cuvelier et al., 2014, 2017; Lelièvre et al., 2017). Finally, electronic tags have been applied to migrating deep-water predators (i.e., sablefish Anoplopoma fimbria individuals reported between the upper subsurface layer and depths down to 1,250 m, with mean depth differences between day and night reaching 250 m; Goetz et al., 2018; Sigler and Echave, 2019), showing distinct patterns that potentially depend on prey availability (i.e., both night ascending and, inversely, night descending). A more comprehensive review of similar case-studies and advances in applications of telemetry technologies in marine ecology was provided by Hussey et al. (2015).
Capturing the Rhythmic Movements of the Deep Scattering Layer
DVMs of the deep scattering layer, comprised mainly of zooplankton and mesopelagic fish, have been extensively reported at a global scale (Hays, 2003; Klevjer et al., 2016). Such rhythmic displacement patterns produce a ubiquitous acoustic signature in the pelagic realm, although their total biomass, upper and lower limits vary across oceanic fronts, depending on climate trends, surface productivity, light penetration, oxygen levels, temperature and water mixing (Aksnes et al., 2017; Proud et al., 2017, 2019; Behrenfeld et al., 2019). Even though they are most commonly limited to depths down to the lower mesopelagic zone (∼1,000 m), DVMs can reportedly extend to several km into abyssal waters (e.g., Natantian decapods in the Mediterranean; Aguzzi and Company, 2010). Traditionally, they are captured as anomalies in the acoustic backscatter signal (due to different reflective properties attributed to the physical differences of animal tissue and seawater and the presence of the swim bladder in the case of fish; Marshall, 1951) by either upward- or downward-facing Acoustic Doppler Current Profilers–ADCPs (Flagg and Smith, 1989; Plueddemann and Pinkel, 1989; Heywood et al., 1991; Ochoa et al., 2013; Bozzano et al., 2014; De Leo et al., 2018) or sonars/echosounders (Barham, 1966; Opdal et al., 2008; Benoit-Bird et al., 2017; Giorli et al., 2018; Van Engeland et al., 2019), as well as with trawl and plankton net surveys (Roe, 1984; Fock et al., 2002; Steinberg et al., 2002; Drazen et al., 2011; Darnis and Fortier, 2014). Remarkably, trawl avoidance behavior has been reported for some mesopelagic fish species which adapted their vertical migrating patterns (Kaartvedt et al., 2012), while there is a practically inevitable sampling bias favoring size and robustness in the deep pelagic zone (Craig et al., 2015). This, in addition to net selectivity, may lead to underestimations of biomass if in situ sampling is not accompanied by remote monitoring methods (which can have their own selectivity limitations nonetheless; Kloser et al., 2016).
Bioluminescence is an ecological trait widely distributed amongst marine organisms (Widder, 2010), from shallow to deep-sea environments (Martini and Haddock, 2017), with massive surface aggregations of bioluminescent organisms even visible from space by satellites (Miller et al., 2005). Biomass dominant taxa found in deep scattering layers such as copepods, euphausiids, gelatinous zooplankton (Herring, 1987) and mesopelagic fishes such as the conspicuous myctophids (Paitio et al., 2016), are known to be bioluminescent. Thus, when mass migrating into deeper layers of the ocean, these species can potentially affect the background intensity of ambient light. Indeed, variability of light intensities has already been recorded over multiannual time-series in the deep ocean using sensors, originally installed with the purpose to study neutrino emissions in the ocean’s interior (Tamburini et al., 2013; Martini et al., 2014; Aguzzi et al., 2017). In general, the presence of bioluminescent organisms at aphotic depths, where there are minimal—if any at all—detectable traces of sunlight, can modify the local ambient light regime by being the strongest (or sole) light source (Cronin et al., 2016), consequently shaping local communities and important functions of the respective ecosystems. For example, bioluminescent organisms and their predators are hypothesized to play a major role in the biological carbon pump, through preferential consumption of luminous particles by high-level consumers. This can affect the sinking rates of the former, their remineralization and their availability in the deeper waters (Tanet et al., 2020) or lead to higher success rates of visual predation for macro-organisms (Vacquié-Garcia et al., 2012).
In the absence of an unequivocal direct relationship between bioluminescence and acoustic backscatter signals (Berge et al., 2012), these variables should ideally be measured in tandem for the study of deep DVMs. As both methods face their own challenges in terms of resolving taxonomic identity, measuring the deep-sea light emission spectrum can be an efficient tool to complement the characterization of the species composition and abundance of the migrating organisms, in a similar way to previous shallow-water applications (Messié et al., 2019). Since the knowledge on the full spectrum of the ecological importance of bioluminescence for vertically migrating groups and for deep-sea benthos is yet to be completed (Martini et al., 2019), we propose a few overarching questions that should direct future long-term ecological monitoring, centered on the extent of deep DVMs:
(1) How to use innovative technologies to monitor deep DVMs in the large, three-dimensional open ocean environment, in the context of benthopelagic coupling? How to couple observations from the water column dimension with now more accessible 2D and 3D video imaging of deep seafloor ecosystems?
(2) Could bioluminescence be used, as a proxy for the extent of DVMs, as well as for large-scale environmental fluctuations linked to climate change and anthropogenic disturbance (e.g., ocean warming, de-oxygenation, ocean acidification and overfishing)?
(3) How to quantify the effect of the mass displacement of bioluminescent organisms, as a component of the migrating deep scattering layer, on the biodiversity and functioning of benthic ecosystems?
Tuning Ocean Monitoring to Catch the Spatiotemporal Scales of Biological Rhythms
With the three-dimensional nature of the marine environment bound to become a central aspect for its conservation (Levin et al., 2018; Aspillaga et al., 2019; Totti et al., 2020), and in order to efficiently track such massive displacements occurring at all depths of the continental margins and the overlying water column volumes (Aguzzi et al., 2011; Rountree et al., 2020), the concept of the geometry of monitoring networks should follow through. New observational technologies are able to detect and quantify the movement of deep scattering layers: neutrino telescopes such as the KM3NeT neutrino telescope network2, presently deployed 40 km offshore south of Toulon (Ligurian Sea) and 100 km offshore southeast of Capo Passero (Ionian Sea); Moored vertical structures equipped with Photo-Multiplier Tubes (PMTs) used as photon counters can pick up photons produced by bioluminescence, for example when animals hit the structure and emit a defensive signal (Priede et al., 2008; Ageron et al., 2011; Tamburini et al., 2013; Craig et al., 2015; Van Haren et al., 2015; Adrián-Martínez et al., 2016; Aguzzi et al., 2017). This setting acts as a relatively passive (i.e., not actively moving) observer of bioluminescence, as animals cross an area permanently occupied by the moored structures and are not reacting to the approach of a potential mobile threat (e.g., towed nets). In that way, any potential bias imposed by a reactionary behavioral control of bioluminescence is expected to be constant in time and across depths. On the other hand, the detection of bioluminescence for communication purposes among animals would be a desirable signal, of ecological significance in the deep sea (alongside sound emissions; Rountree et al., 2012). Time-series of those readings can be produced in real-time, continuously over several years, although, unfortunately, a direct identification of the light-producing organisms is not yet possible. A solution could be the deployment of imaging systems conjointly to PMTs. For example, the use of ultra-low-light imaging technology (Phillips et al., 2016) coupled with measurements from neutrino telescopes will allow cross-linking the bioluminescence light-emission bursts with emitting species at all or most of the mounted PMTs. PMT data can be used both to analyze the waveforms of light bursts, and to extract time-integrated information, such as burst rates. A proposed enhancement is the use of a subset of “ad hoc” PMTs equipped with wavelength filters, to allow spectral analysis of bioluminescent emissions. In that way, taxonomic richness of bioluminescent species could be obtained from imagery, to be contrasted with PMT readings. Different taxa produce different flash types (e.g., signal propagation into the body of animals), which could be used as a morphological trait for their identification (Mazzei et al., 2014).
Such video monitoring could be extended to the deep seabed, for a temporally synchronous and integrated coverage of the whole deep-water column (Aguzzi et al.,2020b,c; Rountree et al., 2020). The main objective would be the detection and quantification of the temporal responses of predators and preys in relation to the rhythmic arrival of the bioluminescent species of the migrating scattering layer (Hays et al., 2010). The deployment of new prototype detectors of the KM3NeT at abyssal Mediterranean depths and covering different depth ranges of the water column (i.e., ORCA; NW basin; ∼3,400 m; strings of ∼150 m length and ARCA; Central basin, ∼2,500 m; strings of ∼650 m length3) will be a powerful tool toward that direction.
Carbon transfer by bioluminescent migrators is a three-dimensional process, as the vertical movement of animals is temporally structured across various oceanic layers, and can be combined with a horizontal displacement. The latter may vary, depending on local circulation patterns which can drag weak swimmers and contribute to plankton dispersal (Hill, 1998; Sato and Benoit-Bird, 2017). Therefore, a spatiotemporally integrated measurement protocol should be executed at appropriate geographical scales and depth ranges, in order to accurately represent this ecosystem service. Accordingly, Remotely Operated Vehicles (ROVs), Autonomous Underwater Vehicles (AUVs), and acoustic backscatter surveys also performed continuously over the 24-h for several days in the same area, should be complementing fixed cameras and benthic crawlers such as Internet Operated Vehicles (IOVs) on the seabed (Aguzzi et al., 2020a). In particular, ongoing actions are aiming to match bioluminescence signals with faunal data extracted from ROV footage obtained during maintenance operations of the telescope tower, which will also be complemented with new imaging data from the expansion of the nearby CREEP-2 cabled observatory (Aguzzi et al., 2013). At the same time, PMT data from the lower floors (i.e., closer to the seafloor) could be compared with richness records proceeding from baited lander surveys in the Central basin area, where the KM3NeT-It telescope is (Linley et al., 2018). A conceptual, minimalistic representation of the monitoring protocol is presented in Figure 1. A recent project focuses on implementing a benthic crawler; BathyBot (Martini et al., 2020b) with video cameras, close to the KM3NeT EMSO-LO site, off Toulon (Ageron et al., 2011; Tamburini et al., 2013). Such approach will represent a suitable asset, allowing the integration of the seabed perspective with the water-column monitoring by a nearby network of photomultipliers. The detection of the presence of animals, the quantification of their abundance and potentially the estimation of their biomass visible in our depth-related sampling windows, would allow the description of relevant behavioral interactions and hence improve our mechanistic understanding of the resulting ecosystem phenotype: the changes in the observed biodiversity on a diel and seasonal basis in the deep-sea (Aguzzi et al., 2020c), based on previous methodologies and permanent mobile platform technologies tested in shallow waters (e.g., at the Obsea cabled observatory; Condal et al., 2012; Del Rio et al., 2020).
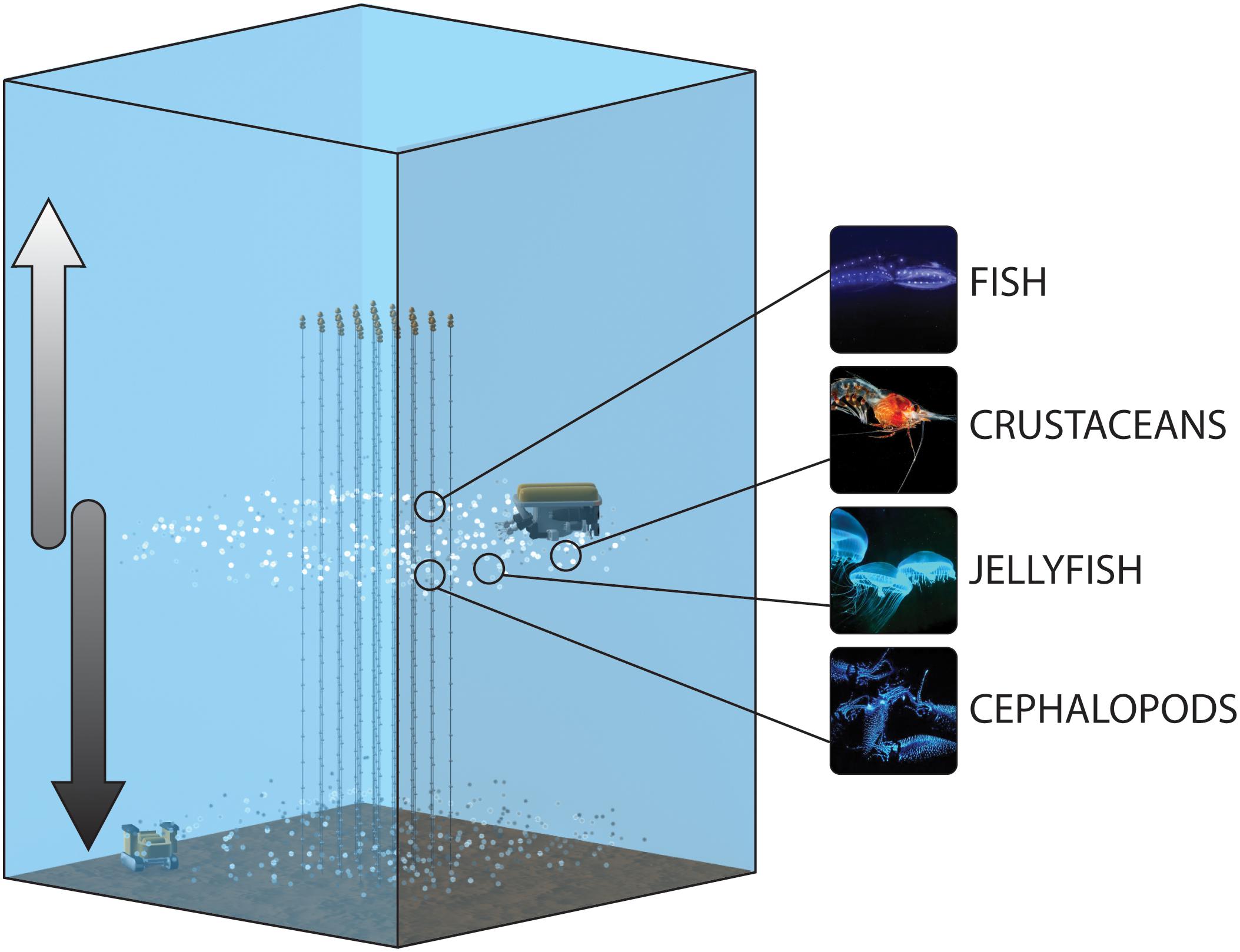
Figure 1. Conceptual schematic of the protocol for the monitoring of the bioluminescent deep-scattering layers, including the strings of the neutrino telescopes bearing the PMT tubes, as well as imaging platforms for the water column (ROV) and seabed (crawler). The arrows represent the descending and ascending of migrators toward the seabed and shallower depths, respectively, which would be depicted as successive peaks in the time-series generated by the distinct platforms.
In the NE Pacific, ∼300 km offshore Canada’s British Columbia, a pathfinder project envisioning the installation of a full-scale neutrino telescope is underway4 (Boehmer et al., 2019; Agostini et al., 2020). The first phase of the project has deployed an initial experiment, STRAW (STRings for Absorption length in Water) with the goal to establish baseline measurements of light attenuation, absorption and scattering at abyssal depths in the NE Pacific (Agostini et al., 2020). Two 150 m long mooring lines were deployed at 2,660 m depth in the Canadian abyssal plain (Cascadia Basin) and connected via ethernet cable with the NEPTUNE observatory (Figure 2). In its second iteration, STRAW-b, a single and substantially longer (450 m) mooring array is now equipped with 10 sensor modules that include a range of PMTs, spectrometers and ultra-low-light cameras that will aid a much greater capability to quantify bioluminescence and possibly assign individual taxa to specific wavelength emission signals (Figure 2). Ongoing data analysis already includes the quantification of temporally diffused vs. intermittent burst bioluminescence signals and their control predominantly by turbulence derived from internal tide frequencies. In addition, modeling efforts are being carried out to study bioluminescence signal response under different turbulent flow regime scenarios and using different taxa as source populations. Finally, the co-location with a standard suit of oceanographic, seismic and biological sensors, will allow for a number of multidisciplinary studies engaging particle physicists, oceanographers and marine ecologists. In particular, the NE Pacific has been subject to a range of environmental shifts in the last decade, including a few marine heat wave anomalies (Kintisch, 2015; Peterson et al., 2017) and systemic de-oxygenation and expansion of its oxygen minimum zone (Whitney et al., 2007; Ross et al., 2020). Having a reliable long-term time-series of bioluminescence could provide another tool to monitor large-scale ecosystem changes in the NE Pacific.
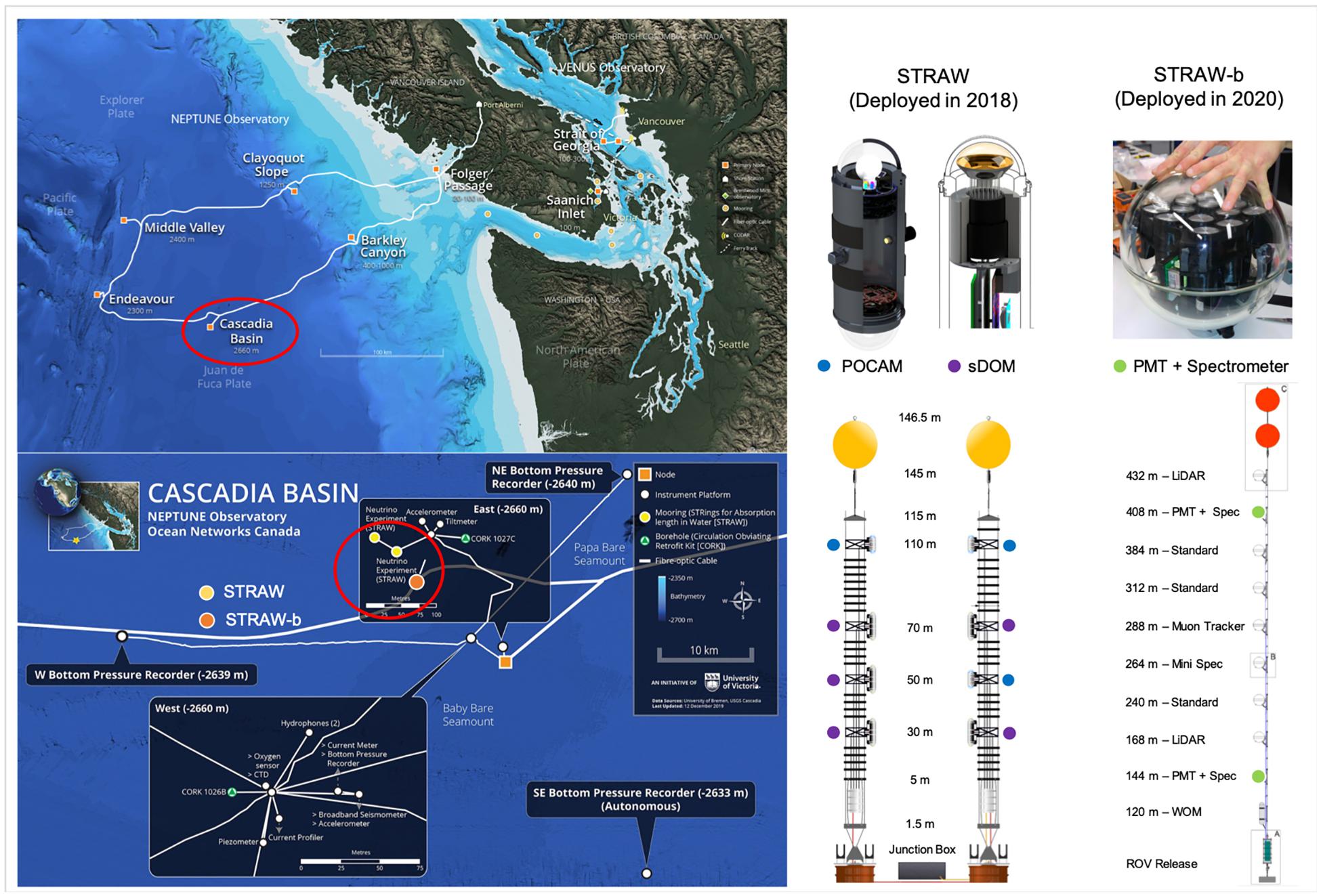
Figure 2. Top left: map of the NEPTUNE cabled observatory installed in the NE Pacific offshore Vancouver Island, Canada. Bottom left: detailed map of the seafloor monitoring infrastructure at the Cascadia Basin observatory node (47.7562°N; 127.7317°W), with exact locations of STRAW and STRAW-b moorings. Top right: examples of sensor modules. POCAM, sDOM, and PMT stand for Precision Optical CAlibration Module, STRAW Digital Optical Module, and Photo-Multiplier Tube, respectively. Bottom right: detailed schematic of the mooring configurations (not to scale), with color symbols indicating where specific bioluminescence-measurement sensors are installed along each mooring.
Implementing such monitoring protocols on a global scale is not a viable option at present, as the cost of the infrastructure and operations would be prohibitive, which in turn could raise the need for the identification of priority regions. These cannot be defined based on purely ecological criteria, rather than being the result of open dialogue between the needs of different scientific communities, taking into account political and logistical issues together with scientific knowledge. Neutrino telescopes (existing or in development) are built in specific locations indicated by the astrophysics community, based on an optimal combination of minimal signal noise at abyssal depths and limitations such as feasibility of access and maintenance operations (e.g., distance from the shore). Similar questions were faced by the marine science community a couple of decades ago, when the first plans to install cabled observatories in the deep sea began to take shape. At present there are more cabled observatories than neutrino telescopes in the ocean, which ultimately makes the latter the limiting factor when it comes to the geographical coverage of the proposed plan. With DVMs and bioluminescence being globally ubiquitous, a combination of such infrastructures set at any region could be utilized to answer the respective ecological questions of relevance, starting from a local level and with the potential to extrapolate to regional-scale phenomena.
Conclusion
• Monitoring the DVMs of shoals of bioluminescent migrators could shed light on the ecological functioning of deep-sea benthic ecosystems and biological connectivity, supporting the need for creative and innovative monitoring protocols.
• Novel infrastructure, such as neutrino telescopes, can help monitor bioluminescent DVMs down to the bathy- and abyssopelagic BBL, providing another asset toward a holistic monitoring network for benthic and water-column ecosystems, alongside current imaging and acoustic methodologies from fixed and mobile platforms.
• With interdisciplinary dialogue among astrophysicists, marine engineers and ecologists, data collection technologies and protocols can be tuned to cross-validate scans of the bioluminescence panoramas with imagery and backscatter data, to resolve the taxonomy of the light-emitting species.
Author Contributions
DC: conceptualization, writing—original draft, and writing—review and editing. NB, SM, GR, and RD: writing—original draft and writing—review and editing. JR and BP: writing—original draft, writing—review and editing, and funding acquisition. MT: writing—original draft, writing—review and editing, and visualization. FD: writing—original draft, writing—review and editing, visualization, and funding acquisition. JA: conceptualization, writing—original draft, writing—review and editing, and funding acquisition. All authors contributed to the article and approved the submitted version.
Funding
This work was developed within the framework of the Tecnoterra (ICM-CSIC/UPC) and the following project activities: ARIM (Autonomous Robotic sea-floor Infrastructure for benthopelagic Monitoring; MartTERA ERA-Net Cofound) and RESBIO (TEC2017-87861-R; Ministerio de Ciencia, Innovación y Universidades; PIs: JR and JA) and with funding from the Spanish government through the ‘Severo Ochoa Centre of Excellence’ accreditation (CEX2019-000928-S). Ocean Networks Canada was funded through Canada Foundation for Innovation-Major Science Initiative Fund 30199 to FD and BP. The STRAW and STRAW-b experiments deployed and connected through the NEPTUNE cabled observatory are supported by the German Research Foundation through grant SFB 1258 “Neutrinos and Dark Matter in Astro- and Particle Physics,” the cluster of excellence “Origin and Structure of the Universe,” and the University of Alberta.
Conflict of Interest
The authors declare that the research was conducted in the absence of any commercial or financial relationships that could be construed as a potential conflict of interest.
Acknowledgments
We would like to thank the NE Pacific neutrino telescope project (P-ONE) team for providing the original schematic of STRAW and STRAW-b moorings. A special acknowledgment is due to the NEMO and ANTARES Consortium, which is providing the framework for the ongoing collaboration. BP and FD wish to thank ONC’s Marine Operations team for at sea and shore support for the installations of STRAW and STRAW-b experiments.
Footnotes
- ^ www.oceannetworks.ca
- ^ https://www.km3net.org/
- ^ https://www.km3net.org/research/physics/
- ^ https://www.pacific-neutrino.org/
References
Adrián-Martínez, S., Aiello, S., Ameli, F., Anghinolfi, M., Ardid, M., Barbarino, G., et al. (2016). Long term monitoring of the optical background in the Capo Passero deep-sea site with the NEMO tower prototype. Eur. Phys. J. C 76:68. doi: 10.1140/epjc/s10052-016-3908-0
Ageron, M., Aguilar, J. A., Samarai, I. A., Albert, A., Ameli, F., André, M., et al. (2011). ANTARES: the first undersea neutrino telescope. Nucl. Instrum. Methods Phys. Res. A 656, 11–38.
Agostini, M., Böhmer, M., Bosma, J., Clark, K., Danninger, M., Fruck, C., et al. (2020). The Pacific Ocean neutrino experiment. Nat. Astron. 4, 913–915. doi: 10.1038/s41550-020-1182-4
Aguzzi, J., Albiez, J., Flögel, S., Godø, O. R., Grimsbø, E., Marini, S., et al. (2020a). A flexible autonomous robotic observatory infrastructure for bentho-pelagic monitoring. Sensors 20:1614. doi: 10.3390/s20061614
Aguzzi, J., Chatzievangelou, D., Company, J. B., Thomsen, L., Marini, S., Bonofiglio, F., et al. (2020b). The potential of video imagery from worldwide cabled observatory networks to provide information supporting fish-stock and biodiversity assessment. ICES J. Mar. Sci. 77, 2396–2410. doi: 10.1093/icesjms/fsaa169
Aguzzi, J., Chatzievangelou, D., Francescangeli, M., Marini, S., Bonofiglio, F., del Rio, J., et al. (2020c). The hierarchic treatment of marine ecological information from spatial networks of benthic platforms. Sensors 20:1751. doi: 10.3390/s20061751
Aguzzi, J., Boon, S., Meredith, P., Company, J. B., Costa, C., Menesatti, P., et al. (2013). The Next Expansion of NEMO-SN1, EMSO Node, by Video Imaging Equipment for the Monitoring of the Local Deep-Sea Communities. Rome: EMSO. European Multidisciplinary Seafloor and water column Observatory (EMSO) Symposium: Challenges and Progress.
Aguzzi, J., and Company, J. B. (2010). Chronobiology of deep-water decapod crustaceans on continental margins. Adv. Mar. Biol. 58, 155–225. doi: 10.1016/B978-0-12-381015-1.00003-4
Aguzzi, J., Company, J. B., Costa, C., Matabos, M., Azzurro, E., Mànuel, A., et al. (2012a). “Challenges to the assessment of benthic populations and biodiversity as a result of rhythmic behaviour: video solutions from cabled observatories,” in Oceanography and Marine Biology: An Annual Review, 50, eds R. N. Gibson, R. J. A. Atkinson, J. D. M. Gordon, and R. N. Hughes (Boca Raton, FL: Taylor & Francis CRC Press), 235–286. doi: 10.1201/b12157
Aguzzi, J., Jamieson, A. J., Fujii, T., Sbragaglia, V., Costa, C., Menesatti, P., et al. (2012b). Shifting feeding behaviour of deep-sea buccinid gastropods at natural and simulated food falls. Mar. Ecol. Prog. Ser. 458, 247–253. doi: 10.3354/meps09758
Aguzzi, J., Company, J. B., Costa, C., Menesatti, P., Garcia, J. A., Bahamon, N., et al. (2011). Activity rhythms in the deep-sea: a chronobiological approach. Front. Biosci. 16:131–150. doi: 10.2741/3680
Aguzzi, J., Costa, C., Furushima, Y., Chiesa, J. J., Company, J. B., Menesatti, P., et al. (2010). Behavioral rhythms of hydrocarbon seep fauna in relation to internal tides. Mar. Ecol. Prog. Ser. 418, 47–56. doi: 10.3354/meps08835
Aguzzi, J., Fanelli, E., Ciuffardi, T., Schirone, A., and Craig, J. KM3NeT-Italia/Nemo Collaboration. (2017). Inertial bioluminescence rhythms at the Capo Passero (KM3NeT-Italia) site, Central Mediterranean Sea. Sci. Rep. 7:44938. doi: 10.1038/srep44938
Aguzzi, J., Fanelli, E., Ciuffardi, T., Schirone, A., De Leo, F. C., Doya, C., et al. (2018). Faunal activity rhythms influencing early community succession of an implanted whale carcass offshore Sagami Bay. Japan. Sci. Rep. 8:11163. doi: 10.1038/s41598-018-29431-5
Aguzzi, J., Puig, P., and Company, J. B. (2009). Hydrodynamic, non-photic modulation of biorhythms in the Norway lobster, Nephrops norvegicus (L.). Deep-Sea Res. I 56, 366–373. doi: 10.1016/j.dsr.2008.10.001
Aguzzi, J., Ramirez-Llodra, E., Telesnicki, G., and Camps, M. (2007). Day-night activity rhythm of the cold seep shrimp Alvinocaris stactophila (Caridea: Alvinocarididae) from the Gulf of Mexico. J. Mar. Biol. Ass. U. K. 87, 1175–1180. doi: 10.1017/S0025315407057311
Aguzzi, J., and Sardà, F. (2008). A history of recent advancements on Nephrops norvegicus behavioral and physiological rhythms. Rev. Fish. Biol. Fish. 18, 235–248. doi: 10.1007/s11160-007-9071-9
Aguzzi, J., Sbragaglia, V., Tecchio, S., Navarro, J., and Company, J. B. (2015). Rhythmic behaviour of marine benthopelagic species and the synchronous dynamics of benthic communities. Deep Sea Res. I 95, 1–11. doi: 10.1016/j.dsr.2014.10.003
Aksnes, D. L., Røstad, A., Kaartvedt, S., Martinez, U., Duarte, C. M., and Irigoien, X. (2017). Light penetration structures the deep acoustic scattering layers in the global ocean. Sci. Adv. 3:e1602468. doi: 10.1126/sciadv.1602468
Allen, S. E., and Durrieu de Madron, X. (2009). A review of the role of submarine canyons in deep-ocean exchange with the shelf. Ocean Sci. 5, 607–620. doi: 10.5194/os-5-607-2009
Aspillaga, E., Safi, K., Hereu, B., and Bartumeus, F. (2019). Modelling the three-dimensional space use of aquatic animals combining topography and Eulerian telemetry data. Methods Ecol. Evol. 10, 1551–1557. doi: 10.1111/2041-210X.13232
Aumont, O., Maury, O., Lefort, S., and Bopp, L. (2018). Evaluating the potential impacts of the diurnal vertical migration by marine organisms on marine biogeochemistry. Global Biogeochem. Cycles 32, 1622–1643. doi: 10.1029/2018GB005886
Bahamon, N., Sardà, F., and Aguzzi, J. (2009). Fuzzy diel pattern in commercial catchability of deep-water continental margin species. ICES J. Mar. Sci. 66, 2211–2218. doi: 10.1093/icesjms/fsp190
Barham, E. G. (1966). Deep scattering layer migration and composition: observations from a diving saucer. Science 151, 1399–1403. doi: 10.1126/science.151.3716.1399
Behrenfeld, M. J., Gaube, P., Della Penna, A., O’Malley, R. T., Burt, W. J., Hu, Y., et al. (2019). Global satellite-observed daily vertical migrations of ocean animals. Nature 576, 257–261. doi: 10.1038/s41586-019-1796-9
Benoit-Bird, K. J., Moline, M. A., and Southall, B. L. (2017). Prey in oceanic sound scattering layers organize to get a little help from their friends. Limnol. Oceanogr. 62, 2788–2798. doi: 10.1002/lno.10606
Berge, J., Båtnes, A. S., Johnsen, G., Blackwell, S. M., and Moline, M. A. (2012). Bioluminescence in the high Arctic during the polar night. Mar. Biol. 159, 231–237. doi: 10.1007/s00227-011-1798-0
Bernardello, R., Cardoso, J. G., Bahamon, N., Donis, D., Marinov, I., and Cruzado, A. (2012). Modelled interannual variability of vertical organic matter export related to phytoplankton bloom dynamics – a case-study for the NW Mediterranean Sea. Biogeosciences 9, 4233–4245. doi: 10.5194/bg-9-4233-2012
Bianchi, D., Stock, C., Galbraith, E. D., and Sarmiento, J. L. (2013). Diel vertical migration: ecological controls and impacts on the biological pump in a one-dimensional ocean model. Global Biochem. Cycles 27, 478–491. doi: 10.1002/gbc.20031
Bicknell, A. W. J., Godley, B. J., Sheehan, E. V., Votier, S. C., and Witt, M. J. (2016). Camera technology for monitoring marine biodiversity and human impact. Front. Ecol. Environ. 14:424–432. doi: 10.1002/fee.1322
Boehmer, M., Bosma, J., Brussow, D., Farmer, L., Fruck, C., Gernhäuser, R., et al. (2019). STRAW (STRings for Absorption length in Water): pathfinder for a neutrino telescope in the deep Pacific Ocean. J. Instrum. 14:02013. doi: 10.1088/1748-0221/14/02/P02013
Bozzano, R., Fanelli, E., Pensieri, S., Picco, P., and Schiano, M. E. (2014). Temporal variations of zooplankton biomass in the Ligurian Sea inferred from long time series of ADCP data. Ocean Sci. 10, 93–105. doi: 10.5194/os-10-93-2014
Brierley, A. S. (2014). Diel vertical migration. Curr. Biol. 24, R1074–R1076. doi: 10.1016/j.cub.2014.08.054
Canals, M., Puig, P., de Madron, X., Heussner, S., Palanques, A., and Fabres, J. (2006). Flushing submarine canyons. Nature 444, 354–357. doi: 10.1038/nature05271
Chatzievangelou, D., Doya, C., Thomsen, L., Purser, A., and Aguzzi, J. (2016). High-frequency patterns in the abundance of benthic species near a cold-seep - an internet operated vehicle application. PLoS One 11:e0163808. doi: 10.1371/journal.pone.0163808
Chauvet, P., Metaxas, A., Hay, A. E., and Matabos, M. (2018). Annual and seasonal dynamics of deep-sea megafaunal epibenthic communities in Barkley Canyon (British Columbia, Canada): a response to climatology, surface productivity and benthic boundary layer variation. Prog. Oceanogr. 169, 89–105. doi: 10.1016/j.pocean.2018.04.002
Chiesa, J. J., Aguzzi, J., García, J. A., Sardà, F., and de la Iglesia, H. O. (2010). Light intensity determines temporal niche switching of behavioral activity in deep-water Nephrops norvegicus (Crustacea: Decapoda). J. Biol. Rhythms 25, 277–287. doi: 10.1177/0748730410376159
Clark, M. R., Consalvey, M., and Rowden, A. A. (2016). Biological Sampling in the Deep Sea. Hoboken, NJ: Wiley Blackwell.
Condal, F., Aguzzi, J., Sardà, F., Nogueras, M., Cadena, J., Costa, C., et al. (2012). Seasonal rhythm in a Mediterranean coastal fish community as monitored by a cabled observatory. Mar. Biol. 159, 2809–2817. doi: 10.1007/s00227-012-2041-3
Costello, M. J., and Chaudhary, C. (2017). Marine biodiversity, biogeography, deep-sea gradients, and conservation. Curr. Biol. 27, R511–R527. doi: 10.1016/j.cub.2017.04.060
Costello, M. J., Cheung, A., and De Hauwere, N. (2010). Topography statistics for the surface and seabed area, volume, depth and slope, of the world’s seas, oceans and countries. Environ. Sci. Technol. 44, 8821–8828. doi: 10.1021/es1012752
Costello, M. J., Wilson, S., and Houlding, B. (2012). Predicting total global species richness using rates of species description and estimates of taxonomic effort. Syst. Biol. 61:871. doi: 10.1055/s-0042-103934
Craig, J., Priede, I. G., Aguzzi, J., Company, J. B., and Jamieson, A. J. (2015). Abundant bioluminescent sources of low-light intensity in the deep Mediterranean Sea and North Atlantic Ocean. Mar. Biol. 162, 1637–1649. doi: 10.1007/s00227-015-2700-2
Cronin, H., Cohen, J., Berge, J., Johnsen, G., and Moline, M. A. (2016). Bioluminescence as an ecological factor during high Arctic polar night. Sci. Rep. 6:36374. doi: 10.1038/srep36374
Cuvelier, D., Legendre, P., Laes, A., Sarradin, P. M., and Sarrazin, J. (2014). Rhythms and community dynamics of a hydrothermal tubeworm assemblage at Main Endeavour field - A multidisciplinary deep-sea observatory approach. PLoS One 9:e96924. doi: 10.1371/journal.pone.0096924
Cuvelier, D., Legendre, P., Laës-Huon, A., Sarradin, P. M., and Sarrazin, J. (2017). Biological and environmental rhythms in (dark) deep-sea hydrothermal ecosystems. Biogeosciences 14, 2955–2977. doi: 10.5194/bg-14-2955-2017
Danovaro, R., Company, J. B., Corinaldesi, C., D’Onghia, G., Galil, B., Gambi, C., et al. (2010). Deep-sea biodiversity in the Mediterranean Sea: the known, the unknown, and the unknowable. PLoS One 5:e11832. doi: 10.1371/journal.pone.0011832
Darnis, G., and Fortier, L. (2014). Temperature, food and the seasonal vertical migration of key arctic copepods in the thermally stratified Amundsen Gulf (Beaufort Sea. Arctic Ocean), J. Plankton Res. 36, 1092–1108. doi: 10.1093/plankt/fbu035
Davies, S., Griffiths, A., and Reimchen, T. E. (2006). Pacific hagfish, Eptatretus stoutii, spotted ratfish, Hydrolagus colliei, and scavenger activity on tethered carrion in subtidal benthic communities off Western Vancouver Island. Can. Field Nat. 120, 363–366. doi: 10.22621/cfn.v120i3.329
Davison, P. C., Checkley, D. M. Jr., Koslowa, J. A., and Barlow, J. (2013). Carbon export mediated by mesopelagic fishes in the northeast Pacific Ocean. Prog. Oceanogr. 116, 14–30. doi: 10.1016/j.pocean.2013.05.013
De Leo, F. C., Ogata, B., Sastri, A. R., Heesemann, M., Mihály, S., Galbraith, M., et al. (2018). High-frequency observations from a deep-sea cabled observatory reveal seasonal overwintering of Neocalanus spp. in Barkley Canyon, NE Pacific: insights into particulate organic carbon flux. Prog. Oceanogr. 169, 120–137.
Del Rio, J., Sarria, D., Aguzzi, J., Masmitja, I., Carandell, M., Olive, J., et al. (2020). Obsea: a decadal balance for a cabled observatory deployment. IEEE Access 8, 33163–33177. doi: 10.1109/ACCESS.2020.2973771
Doya, C., Aguzzi, J., Pardo, M., Matabos, M., Company, J. B., Costa, C., et al. (2014). Diel behavioral rhythms in sablefish (Anoplopoma fimbria) and other benthic species as recorded by the deep-sea cabled observatories in Barkley canyon (NEPTUNE-Canada). J. Mar. Syst. 130, 69–78. doi: 10.1016/j.jmarsys.2013.04.003
Drazen, J. C., De Forest, L. G., and Domokos, R. (2011). Micronekton abundance and biomass in Hawaiian waters as influenced by seamounts, eddies, and the moon. Deep Sea Res. I 58, 557–566. doi: 10.1016/j.dsr.2011.03.002
Drazen, J. C., Smith, C. R., Gjerde, K. M., Haddock, S. H. D., Carter, G. S., Choy, C. A., et al. (2020). Opinion: midwater ecosystem must be considered when evaluating environmental risks of deep-sea mining. Proc. Natl. Acad. Sci. U.S.A 117, 17455–17460.
Drazen, J. C., and Sutton, T. T. (2017). Dining in the deep: the feeding ecology of deep-sea fishes. Annu. Rev. Mar. Sci. 9, 337–366. doi: 10.1146/annurev-marine-010816-060543
Flagg, C. N., and Smith, S. L. (1989). On the use of the acoustic Doppler current profiler to measure zooplankton abundance. Deep Sea Res. A 36, 455–474. doi: 10.1016/0198-0149(89)90047-2
Fock, H. O., Matthiessen, B., Zidowitz, H., and Von Westernhagen, H. (2002). Diel and habitat-dependent resource utilisation by deep-sea fishes at the Great Meteor seamount: niche overlap and support for the sound scattering layer interception hypothesis. Mar. Ecol. Prog. Ser. 244, 219–233. doi: 10.3354/meps244219
Gage, J. D., and Tyler, P. A. (1991). Deep-Sea Biology: A Natural History of Organisms at the Deep Sea Floor. Cambridge: Cambridge University Press.
Gardner, W. D., Tucholke, B. E., Richardson, M. J., and Biscaye, P. E. (2017). Benthic storms, nepheloid layers, and linkage with upper ocean dynamics in the western North Atlantic. Mar. Geol. 385, 304–327. doi: 10.1016/j.margeo.2016.12.012
Gerkema, M. P., Davies, W. I., Foster, R. G., Menaker, M., and Hut, R. A. (2013). The nocturnal bottleneck and the evolution of activity patterns in mammals. Proc. R. Soc. B 280:20130508. doi: 10.1098/rspb.2013.0508
Gillard, B., Purkiani, K., Chatzievangelou, D., Vink, A., Iversen, M. H., and Thomsen, L. (2019). Physical and hydrodynamic properties of deep sea mining-generated, abyssal sediment plumes in the Clarion Clipperton Fracture Zone (eastern-central Pacific). Elem. Sci. Anth. 7:5. doi: 10.1525/elementa.343
Giorli, G., Drazen, J. C., Neuheimer, A. B., Copeland, A., and Au, W. W. L. (2018). Deep sea animal density and size estimated using a Dual-frequency IDentification SONar (DIDSON) offshore the island of Hawaii. Prog. Oceanogr. 160, 155–166. doi: 10.1016/j.pocean.2018.01.002
Gjøsæter, H., Wiebe, P. H., Knutsen, T., and Ingvaldsen, R. B. (2017). Evidence of diel vertical migration of mesopelagic sound-scattering organisms in the Arctic. Front. Mar. Sci. 4:332. doi: 10.3389/fmars.2017.00332
Goetz, F. W., Jasonowicz, A. J., and Roberts, S. B. (2018). What goes up must come down: diel vertical migration in the deep-water sablefish (Anoplopoma fimbria) revealed by pop-up satellite archival tags. Fish. Oceanogr. 27, 127–142. doi: 10.1111/fog.12239
Gordon, J. D. M., Bergstad, O. A., Figueiredo, I., and Menezes, G. (2003). Deep-water fisheries of the Northeast Atlantic: I. Description and current trends. J. Northw. Atl. Fish. Sci. 31, 137–150.
Griffiths, J. R., Francisco, M. K., Nascimento, J. A., Tamelander, T., Törnroos, A., Bonaglia, S., et al. (2017). The importance of benthic–pelagic coupling for marine ecosystem functioning in a changing world. Glob. Change Biol. 23, 2179–2196. doi: 10.1111/gcb.13642
Gross, T. F., and Williams, A. J.III. (1991). Characterization of deep-sea storms. Mar. Geol. 99, 281–301. doi: 10.1016/0025-3227(91)90045-6
Haddock, S. H. D., Christianson, L. M., Francis, W. R., Martini, S., Dunn, C. W., Pugh, P. R., et al. (2017). Insights into the biodiversity, behavior, and bioluminescence of deep-sea organisms using molecular and maritime technology. Oceanography 30, 38–47. doi: 10.5670/oceanog.2017.422
Haddock, S. H. D., Moline, M. A., and Case, J. F. (2010). Bioluminescence in the sea. Ann. Rev. Mar. Sci. 2, 443–493. doi: 10.1146/annurev-marine-120308-081028
Hart, T. D., Clemons, J. E., Wakefield, W. W., and Heppell, S. S. (2010). Day and night abundance, distribution, and activity patterns of demersal fishes on Heceta Bank. Oregon. Fish. Bull. 108, 466–477.
Hays, G. C. (2003). A review of the adaptive significance and ecosystem consequences of zooplankton diel vertical migrations. Hydrobiologia 503, 163–170. doi: 10.1023/B:HYDR.0000008476.23617.b0
Hays, G. C., Ferreira, L. C., Sequeira, A. M. M., Meekan, M. G., Duarte, C. M., Bailey, H., et al. (2010). Key questions in marine megafauna movement ecology. Trends Ecol. Evol. 31, 463–475. doi: 10.1016/j.tree.2016.02.015
Helm, B., Ben-Shlomo, R., Sheriff, M. J., Hut, R. A., Foster, R., Barnes, B. M., et al. (2013). Annual rhythms that underlie phenology: biological time-keeping meets environmental change. Proc. R. Soc. B 280:20130016. doi: 10.1098/rspb.2013.0016
Herring, P. J. (1987). Systematic distribution of bioluminescence in living organisms. J. Biolumin. Chemilumin. 1, 147–163. doi: 10.1002/bio.1170010303
Hessler, R. R., and Sanders, H. L. (1967). Faunal diversity in the deep-sea. Deep-Sea Res. I 14, 65–78. doi: 10.1016/0011-7471(67)90029-0
Heywood, K. J., Scrope-Howe, S., and Barton, E. D. (1991). Estimation of zooplankton abundance from shipborne ADCP backscatter. Deep-Sea Res. A 38, 677–691. doi: 10.1016/0198-0149(91)90006-2
Hidalgo, M., and Browman, H. I. (2019). Developing the knowledge base needed to sustainably manage mesopelagic resources. ICES J. Mari. Sci. 76, 609–615. doi: 10.1093/icesjms/fsz067
Hill, A. E. (1998). Diel vertical migration in stratified tidal flows: implications for plankton dispersal. J. Mar. Res. 56, 1069–1096. doi: 10.1357/002224098765173464
Hollister, C. D., and McCave, I. N. (1984). Sedimentation under deep-sea storms. Nature 309, 220–222. doi: 10.1038/309220a0
Houpert, L., Durrieu de Madron, X., Testor, P., Bosse, A., D’Ortenzio, F., Bouin, M. N., et al. (2016). Observations of open-ocean deep convection in the northwestern Mediterranean Sea: seasonal and interannual variability of mixing and deep water masses for the 2007-2013 period. J. Geophys. Res. Oceans 121, 8139–8171. doi: 10.1002/2016JC011857
Hussey, N. E., Kessel, S. T., Aarestrup, K., Cooke, S. J., Cowley, P. D., Fisk, A. T., et al. (2015). Aquatic animal telemetry: a panoramic window into the underwater world. Science 348:1255642. doi: 10.1126/science.1255642
Hut, R. A., Paolucci, S., Dor, R., Kyriacou, C. P., and Daan, S. (2013). Latitudinal clines: an evolutionary view on biological rhythms. Proc. R. Soc. B 280:20130433. doi: 10.1098/rspb.2013.0433
Irigoien, X., Klevjer, T. A., Røstad, A., Martinez, U., Boyra, G., Acuña, J. L., et al. (2014). Large mesopelagic fishes biomass and trophic efficiency in the open ocean. Nat. Commun. 5:3271. doi: 10.1038/ncomms4271
Johnsen, S., Frank, T. M., Haddock, S. H. D., Widder, E. A., and Messing, C. G. (2012). Light and vision in the deep-sea benthos: I. Bioluminescence at 500-1000 m depth in the Bahamian Islands. J. Exp. Biol. 215, 3335–3343. doi: 10.1242/jeb.072009
Kaartvedt, S., Staby, A., and Aksnes, D. L. (2012). Efficient trawl avoidance by mesopelagic fishes causes large underestimation of their biomass. Mar. Ecol. Prog. Ser. 456, 1–6. doi: 10.3354/meps09785
Kaltenberg, A., Biggs, D., and DiMarco, S. (2007). Deep scattering layers of the Northern Gulf of Mexico observed with a shipboard 38-kHz Acoustic Doppler Current Profiler. Gulf Mex. Sci. 25:1. doi: 10.18785/goms.2502.01
Kao, S. J., Dai, M., Selvaraj, K., Zhai, Z., Cai, P., Chen, S. N., et al. (2010). Cyclone-driven deep sea injection of freshwater and heat by hyperpycnal flow in the subtropics. Geophys. Res. Lett. 37:L21702. doi: 10.1029/2010GL044893
Kelly, T. B., Davison, P. C., Goericke, R., Landry, M. R., Ohman, M. D., and Stukel, M. R. (2019). The importance of mesozooplankton diel vertical migration for sustaining a mesopelagic food web. Front. Mar. Sci. 6:508. doi: 10.3389/fmars.2019.00508
Kintisch, E. (2015). ‘The Blob’ invades Pacific, flummoxing climate experts. Science 348, 17–18. doi: 10.1126/science.348.6230.17
Klevjer, T. A., Irigoien, X., Røstad, A., Fraile-Nuez, E., Benítez-Barrios, V. M., and Kaartvedt, S. (2016). Large scale patterns in vertical distribution and behaviour of mesopelagic scattering layers. Sci. Rep. 6:19873. doi: 10.1038/srep19873
Kloser, R. J., Ryan, T. E., Keith, G., and Gershwin, L. (2016). Deep-scattering layer, gas-bladder density, and size estimates. ICES J. Mar. Sci. 73, 2037–2048. doi: 10.1093/icesjms/fsv257
Koeve, W., Pollehne, F., Oschlies, A., and Zeitzschel, B. (2002). Storm-induced convective export of organic matter during spring in the Northeast Atlantic Ocean. Deep-Sea Res. I 49, 1431–1444. doi: 10.1016/S0967-0637(02)00022-5
Lambert, C., Laran, S., David, L., Dorémus, G., Pettex, E., Van Canneyt, O., et al. (2017a). How does ocean seasonality drive habitat preferences of highly mobile top predators? Part I: the north-western Mediterranean Sea. Deep Sea Res. II 141, 115–132. doi: 10.1016/j.dsr2.2016.06.012
Lambert, C., Pettex, E., Dorémus, G., Laran, S., Stéphan, E., Van Canneyt, O., et al. (2017b). How does ocean seasonality drive habitat preferences of highly mobile top predators? Part II: the eastern North-Atlantic. Deep Sea Res. II 141, 133–154. doi: 10.1016/j.dsr2.2016.06.011
Lelièvre, Y., Legendre, P., Matabos, M., Mihály, S., Lee, R. W., Sarradin, P. M., et al. (2017). Astronomical and atmospheric impacts on deep-sea hydrothermal vent invertebrates. Proc. R. Soc. B 284:20162123. doi: 10.1098/rspb.2016.2123
Levin, L. A., and Le Bris, N. (2015). The deep ocean under climate change. Science 350, 766–768. doi: 10.1126/science.aad0126
Levin, L. A., and Sibuet, M. (2012). Understanding continental margin biodiversity: a new imperative. Annu. Rev. Mar. Sci. 4, 79–112. doi: 10.1146/annurev-marine-120709-142714
Levin, L. A., Sibuet, M., Gooday, A. J., Smith, C. R., and Vanreusel, A. (2010). The roles of habitat heterogeneity in generating and maintaining biodiversity on continental margins: an introduction. Mar. Ecol. 31, 1–5. doi: 10.1111/j.1439-0485.2009.00358.x
Levin, N., Kark, S., and Danovaro, R. (2018). Adding the third dimension to marine conservation. Conserv. Lett. 11:e12408. doi: 10.1111/conl.12408
Linley, T. D., Craig, J., Jamieson, A. J., and Priede, I. G. (2018). Bathyal and abyssal demersal bait-attending fauna of the Eastern Mediterranean Sea. Mar. Biol. 165:159. doi: 10.1007/s00227-018-3413-0
Longhurst, A. R., and Harrison, W. G. (1988). Vertical nitrogen flux from the oceanic photic zone by diel migrant zooplankton and nekton. Deep-Sea Res. A 35, 881–889. doi: 10.1016/0198-0149(88)90065-9
Marshall, N. B. (1951). Bathypelagic fishes as sound scatterers in the ocean. J. Mar. Res. 10, 1–17.
Martin, A., Boyd, P., Buesseler, K., Cetinic, I., Claustre, H., Giering, S., et al. (2020). Study the twilight zone before it is too late. Nature 580, 26–28. doi: 10.1038/d41586-020-00915-7
Martini, S., and Haddock, S. (2017). Quantification of bioluminescence from the surface to the deep sea demonstrates its predominance as an ecological trait. Sci. Rep. 7:45750. doi: 10.1038/srep45750
Martini, S., Kuhnz, L., Mallefet, J., and Haddock, S. H. D. (2019). Distribution and quantification of bioluminescence as an ecological trait in the deep sea benthos. Sci. Rep. 9:14654. doi: 10.1038/s41598-019-50961-z
Martini, S., Nerini, D., and Tamburini, C. (2014). Relation between deep bioluminescence and oceanographic variables: a statistical analysis using time–frequency decompositions. Prog. Oceanogr. 127, 117–128. doi: 10.1016/j.pocean.2014.07.003
Martini, S., Schultz, D. T., Lundsten, L., and Haddock, S. H. D. (2020a). Bioluminescence in an undescribed species of carnivorous sponge (Cladorhizidae) from the deep sea. Front. Mar. Sci. 7:576476. doi: 10.3389/fmars.2020.576476
Martini, S., Tamburini, C., Gojak, C., Aguzzi, J., Arnaubec, A., Barnes-Davin, L., et al. (2020b). “BathyBot – a Deep-sea Crawler to See the Unseen in the NW Mediterranean Sea,” in Conference Abstract IS34A-3344: Ocean Sciences Meeting 2020, San Diego, CA: AGU.
Mat, A. M. (2019). Chronobiology and the design of marine biology experiments. ICES J. Mar. Sci. 76, 60–65. doi: 10.1093/icesjms/fsy131
Matabos, M., Bui, A. O. V., Mihály, S., Aguzzi, J., Juniper, S. K., and Ajayamohan, R. S. (2014). High-frequency study of epibenthic megafaunal community dynamics in Barkley Canyon: a multi-disciplinary approach using the NEPTUNE Canada network. J. Mar. Syst. 130, 56–68. doi: 10.1016/j.jmarsys.2013.05.002
Mazzei, L., Marini, S., Craig, J., Aguzzi, J., Fanelli, E., and Priede, I. G. (2014). “Automated video imaging system for counting deep-sea bioluminescence organisms events,” in 2014 ICPR Workshop on Computer Vision for Analysis of Underwater Imagery, Stockholm: IEEE, 57–64. doi: 10.1109/CVAUI.2014.15
McClain, C. R., and Barry, J. P. (2010). Habitat heterogeneity, disturbance, and productivity work in concert to regulate biodiversity in deep submarine canyons. Ecology 91, 964–976. doi: 10.1890/09-0087.1
Meccia, V. L., Borghini, M., and Sparnocchia, S. (2015). Abyssal circulation and hydrographic conditions in the Western Ionian Sea during Spring–Summer 2007 and Autumn–Winter 2007–2008. Deep Sea Res. I 104, 26–40. doi: 10.1016/j.dsr.2015.06.007
Mercier, A., Sun, Z., Baillon, S., and Hamel, J. F. (2011). Lunar rhythms in the deep sea: evidence from the reproductive periodicity of several marine invertebrates. J. Biol. Rhythms 26, 82–86. doi: 10.1177/0748730410391948
Messié, M., Shulman, I., Martini, S., and Haddock, S. H. D. (2019). Using fluorescence and bioluminescence sensors to characterize auto- and heterotrophic plankton communities. Prog. Oceanogr. 171, 76–92. doi: 10.1016/j.pocean.2018.12.010
Miller, S. D., Haddock, S. H. D., Elvidge, C. D., and Lee, T. F. (2005). Detection of a bioluminescent milky sea from space. Proc. Natl. Acad. Sci. U.S.A. 102, 14181–14184. doi: 10.1073/pnas.0507253102
Mora, C., Tittensor, D. P., Adl, S., Simpson, A. G. B., and Worm, B. (2011). How many species are there on earth and in the ocean? PLoS Biol. 9:e1001127. doi: 10.1371/journal.pbio.1001127
Naylor, E. (2005). Chronobiology: implications for marine resource exploitation and management. Sci. Mar. 69, 157–167. doi: 10.3989/scimar.2005.69s1157
Nealson, K. H., Arneson, A. C., and Huber, M. E. (1986). Identification of marine organisms using kinetic and spectral properties of their bioluminescence. Mar. Biol. 91, 77–83. doi: 10.1007/BF00397573
Nuñez, J. D., Sbragaglia, V., García, J. A., Company, J. B., and Aguzzi, J. (2016). First laboratory insight on the behavioral rhythms of the bathyal crab Geryon longipes. Deep Sea Res. I 116, 165–173. doi: 10.1016/j.dsr.2016.08.007
Ochoa, J., Maske, H., Sheinbaumc, J., and Candela, J. (2013). Diel and lunar cycles of vertical migration extending to below 1000 m in the ocean and the vertical connectivity of depth-tiered populations. Limnol. Oceanogr. 58, 1207–1214. doi: 10.4319/lo.2013.58.4.1207
Opdal, A. F., Godø, O. R., Bergstad, O. A., and Fiksen, Ø (2008). Distribution, identity, and possible processes sustaining meso- and bathypelagic scattering layers on the northern Mid-Atlantic Ridge. Deep Sea Res. II 55, 45–58. doi: 10.1016/j.dsr2.2007.09.002
Osterloff, J., Nilssen, I., Järnegren, J., Van Engeland, T., Buhl-Mortensen, P., and Nattkemper, T. W. (2019). Computer vision enables short-and long-term analysis of Lophelia pertusa polyp behaviour and colour from an underwater observatory. Sci. Rep. 9:6578. doi: 10.1038/s41598-019-41275-1
Osterloff, J., Nilssen, I., and Nattkemper, T. W. (2016). A computer vision approach for monitoring the spatial and temporal shrimp distribution at the LoVe observatory. Methods Oceanogr. 15, 114–128. doi: 10.1016/j.mio.2016.03.002
Paitio, J., Oba, Y., and Meyer-Rochow, V. B. (2016). “Bioluminescent fishes and their eyes,” in Luminescence - An Outlook on the Phenomena and Their Applications, ed. J. Thirumalai (Rijeka: IntechOpen), 297–332. doi: 10.5772/65385
Palanques, A., Puig, P., Latasa, M., and Scharek, R. (2009). Deep sediment transport induced by storms and dense shelf-water cascading in the northwestern Mediterranean basin. Deep Sea Res. I 56, 425–434. doi: 10.1016/j.dsr.2008.11.002
Peterson, W. T., Fisher, J. L., Strub, P. T., Du, X., Risien, C., Peterson, J., et al. (2017). The pelagic ecosystem in the Northern California Current off Oregon during the 2014–2016 warm anomalies within the context if the past 20 years. J. Geophys. Res. Oceans 122, 7267–7290. doi: 10.1002/2017JC012952
Phillips, B. T., Grubber, D. F., Vasan, G., Roman, C. N., Pieribone, V. A., and Sparks, J. S. (2016). Observations of in situ deep-sea marine bioluminescence with a high-speed, high-resolution sCMOS camera. Deep Sea Res. I 111, 102–109. doi: 10.1016/j.dsr.2016.02.012
Plueddemann, A. J., and Pinkel, R. (1989). Characterization of the patterns of diel migration using a doppler sonar. Deep-Sea Res. A 36, 509–530. doi: 10.1016/0198-0149(89)90003-4
Priede, I. G., Jamieson, A., Heger, A., Craig, J., and Zuur, A. F. (2008). The potential influence of bioluminescence from marine animals on a deep-sea underwater neutrino telescope array in the Mediterranean Sea. Deep Sea Res. I 55, 1474–1483. doi: 10.1016/j.dsr.2008.07.001
Proud, R., Cox, M. J., and Brierley, A. S. (2017). Biogeography of the global ocean’s mesopelagic zone. Curr. Biol. 27, 113–119. doi: 10.1016/j.cub.2016.11.003
Proud, R., Handegard, N. O., Kloser, R. J., Cox, M. J., and Brierley, A. S. (2019). From siphonophores to deep scattering layers: uncertainty ranges for the estimation of global mesopelagic fish biomass. ICES J. Mar. Sci. 76, 718–733. doi: 10.1093/icesjms/fsy037
Puig, P., Durrieu de Madron, X., Salat, J., Schroeder, K., Martín, J., Karageorgis, A. P., et al. (2013a). Thick bottom nepheloid layers in the western Mediterranean generated by deep dense shelf water cascading. Prog. Oceanogr. 111, 1–23. doi: 10.1016/j.pocean.2012.10.003
Puig, P., Greenan, B. J. W., Li, M. Z., Prescott, R. H., and Piper, D. J. W. (2013b). Sediment transport processes at the head of Halibut Canyon, eastern Canada margin: an interplay between internal tides and dense shelf-water cascading. Mar. Geol. 341, 14–28. doi: 10.1016/j.margeo.2013.05.004
Purser, A. (2015). A time series study of Lophelia pertusa and reef megafauna responses to drill cuttings exposure on the Norwegian margin. PLoS One 10:e0134076. doi: 10.1371/journal.pone.0134076
Ramirez-Llodra, E., Brandt, A., Danovaro, R., De Mol, B., Escobar, E., German, C. R., et al. (2010). Deep, diverse and definitely different: unique attributes of the world’s largest ecosystem. Biogeosciences 7, 2851–2899. doi: 10.5194/bg-7-2851-2010
Rex, M. A., Etter, R. J., Morris, J. S., Crouse, J., McClain, C. R., Johnson, N. A., et al. (2006). Global bathymetric patterns of standing stock and body size in the deep-sea benthos. Mar. Ecol. Prog. Ser. 317, 1–8. doi: 10.3354/meps317001
Roe, H. S. J. (1984). The diel migrations and distributions within a Mesopelagic community in the North East Atlantic. 2. Vertical migrations and feeding of Mysids and decapod Crustacea. Prog. Oceanogr. 13, 269–318. doi: 10.1016/0079-6611(84)90011-9
Ross, T., Du Preez, C., and Ianson, D. (2020). Rapid deep ocean deoxygenation and acidification threaten life on Northeast Pacific seamounts. Glob. Change Biol. 26, 6424–6444. doi: 10.1111/gcb.15307
Rountree, R. A., Aguzzi, J., Marini, S., Fanelli, E., De Leo, F. C., Del Rio, J., et al. (2020). “Towards an optimal design for ecosystem level ocean observatories,” in Oceanography and Marine Biology: An Annual Review, 58, eds S. J. Hawkins, A. L. Allcock, A. E. Bates, A. J. Evans, L. B. Firth, C. D. McQuaid, et al. (London: Taylor & Francis CRC Press), 79–106. doi: 10.1201/9780429351495-2
Rountree, R. A., Juanes, F., Goudey, C. A., and Ekstrom, K. E. (2012). “Is biological sound production important in the deep sea?,” in The Effects of Noise on Aquatic Life. Advances in EXPERIMENTAL Medicine and Biology, 730, eds A. N. Popper and A. Hawkins (New York, NY: Springer), 181–183. doi: 10.1007/978-1-4419-7311-5_41
Rubino, A., Falcini, F., Zanchettin, D., Bouche, V., Salusti, E., Bensi, M., et al. (2012). Abyssal undular vortices in the Eastern Mediterranean basin. Nat. Commun. 3:834. doi: 10.1038/ncomms1836
Sardà, F., and Aguzzi, J. (2012). A review of burrow counting as an alternative to other typical methods of assessment of Norway lobster populations. Rev. Fish Biol. Fishe. 22, 409–422. doi: 10.1007/s11160-011-9242-6
Sardà, F., Cartes, J. E., and Company, J. B. (1994). Spatio-temporal variations in megabenthos abundance in three different habitats of the Catalan deep-sea (Western Mediterranean). Mar. Biol. 120, 211–219. doi: 10.1007/BF00349681
Sato, M., and Benoit-Bird, K. J. (2017). Spatial variability of deep scattering layers shapes the Bahamian mesopelagic ecosystem. Mar. Ecol. Prog. Ser. 580, 69–82. doi: 10.3354/meps12295
Sbragaglia, V., García, J. A., Chiesa, J. J., and Aguzzi, J. (2015). Effect of simulated tidal currents on the burrow emergence rhythms of the Norway lobster (Nephrops norvegicus). Mar. Biol. 162, 2007–2016. doi: 10.1007/s00227-015-2726-5
Sbragaglia, V., Leiva, D., Arias, A., García, J. A., Aguzzi, J., and Breithaupt, T. (2017). Fighting over burrows: the emergence of dominance hierarchies in the Norway lobster (Nephrops norvegicus). J. Exp. Biol. 220, 4624–4633. doi: 10.1242/jeb.165969
Schmidt, K., Atkinson, A., Steigenberger, S., Fielding, S., Lindsay, M. C. M., Pond, D. W., et al. (2011). Seabed foraging by Antarctic krill: implications for stock assessment, bentho-pelagic coupling, and the vertical transfer of iron. Limnol. Oceanogr. 56, 1411–1428. doi: 10.4319/lo.2011.56.4.1411
Schroeder, K., Chiggiato, J., Bryden, L., Borghini, M., and Ben Ismail, S. (2016). Abrupt climate shift in the Western Mediterranean Sea. Sci. Rep. 6:23009. doi: 10.1038/srep23009
Seki, M. P., and Polovina, J. J. (2019). “Ocean Gyre Ecosystems,” in Encyclopedia of Ocean Sciences, 3rd Edn, eds J. K. Cochran, H. J. Bokuniewicz, and P. L. Yager (London: Elsevier Academic Press), 753–758. doi: 10.1016/B978-0-12-409548-9.11331-4
Sigler, M. F., and Echave, K. B. (2019). Diel vertical migration of sablefish (Anoplopoma fimbria). Fish. Oceanogr. 28, 517–531. doi: 10.1111/fog.12428
Smith, C. R., Tunnicliffe, V., Colaço, A., Drazen, J. C., Gollner, S., Levin, L. A., et al. (2020). Deep-sea misconceptions cause underestimation of seabed-mining impacts. Trends Ecol. Evol. 35, 853–857. doi: 10.1016/j.tree.2020.07.002
Smith, K. L. Jr., Sherman, A. D., McGill, P. R., Henthorn, R. G., Ferreira, J., and Huffard, C. L. (2017). Evolution of monitoring an abyssal time-series station in the northeast Pacific over 28 years. Oceanography 30:7281. doi: 10.5670/oceanog.2017.425
Snelgrove, P. V. R. (2016). An ocean of discovery: biodiversity beyond the census of marine life. Plant. Med. 82, 790–799.
Snelgrove, P. V. R., Soetaert, K., Solan, M., Thrush, S., Wei, C. L., Danovaro, R., et al. (2017). Global carbon cycling on a heterogeneous seafloor. Trends Ecol. Evol. 33, 96–105. doi: 10.1016/j.tree.2017.11.004
Srivastava, G., Chakraborty, A., and Nanjundiah, R. S. (2020). Multidecadal variations in ENSO-Indian summer monsoon relationship at sub-seasonal timescales. Theor. Appl. Climatol. 140, 1299–1314. doi: 10.1007/s00704-020-03122-6
St. John, M. A., Borja, A., Chust, G., Heath, M., Grigorov, I., Mariani, P., et al. (2016). A dark hole in our understanding of marine ecosystems and their services: perspectives from the mesopelagic community. Front. Mar. Sci. 3:31. doi: 10.3389/fmars.2016.00031
Steinberg, D. K., Goldthwait, S. A., and Hansell, D. A. (2002). Zooplankton vertical migration and the active transport of dissolved organic and inorganic nitrogen in the Sargasso Sea. Deep Sea Res. I 49, 1445–1461. doi: 10.1016/S0967-0637(02)00037-7
Steinberg, D. K., Van Moy, B. A. S., Buesseler, K. O., Boyd, P. W., Kobari, T., and Karl, D. M. (2008). Bacterial vs. zooplankton control of sinking particle flux in the ocean’s twilight zone. Limnol. Oceanogr. 53, 1327–1338. doi: 10.4319/lo.2008.53.4.1327
Sweetman, A. K., Thurber, A. R., Smith, C. R., Levin, L. A., Mora, C., Wei, C. L., et al. (2017). Major impacts of climate change on deep-sea benthic ecosystems. Elem. Sci. Anth. 5:4. doi: 10.1525/elementa.203
Talley, L. D., Pickard, G. L., Emery, W. J., and Swift, J. H. (2011). Descriptive Physical Oceanography: an Introduction. London: Academic Press.
Tamburini, C., Canals, M., Durrieu de Madron, X., Houpert, L., Lefèvre, D., Martini, S., et al. (2013). Deep-sea bioluminescence blooms after dense water formation at the ocean surface. PLoS One 8:e67523. doi: 10.1371/journal.pone.0067523
Tanet, L., Martini, S., Casalot, L., and Tamburini, C. (2020). Reviews and syntheses: bacterial bioluminescence – ecology and impact in the biological carbon pump. Biogeosciences 17, 3757–3778. doi: 10.5194/bg-17-3757-2020
Tessmar-Raible, K., Raible, F., and Arboleda, E. (2011). Another place, another timer: marine species and the rhythms of life. Bioessays 33, 165–172. doi: 10.1002/bies.201000096
Thomsen, L., Aguzzi, J., Costa, C., De Leo, F., Ogston, A., and Purser, A. (2017). The oceanic biological pump: rapid carbon transfer to depth at continental margins during winter. Sci. Rep. 7:10763. doi: 10.1038/s41598-017-11075-6
Thurber, A. R., Sweetman, A. K., Narayanaswamy, B. E., Jones, D. O. B., Ingels, J., and Hansman, R. L. (2014). Ecosystem function and services provided by the deep sea. Biogeosciences 11, 3941–3963. doi: 10.5194/bg-11-3941-2014
Totti, C., Accoroni, S., Barucca, M., Bianchelli, S., Biscotti, M. A., Calcinai, B., et al. (2020). “Marine Biology. Biodiversity and functioning of marine ecosystems: scientific advancements and new perspectives for preserving marine life,” in The First Outstanding 50 Years of “Università Politecnica delle Marche”, eds S. Longhi, A. Monteriù, A. Freddi, L. Aquilanti, M. G. Ceravolo, O. Carnevali, et al. (Cham: Springer), 447–462.
Trenkel, V. M., Le Loc’h, F., and Rochet, M. J. (2007). Small-scale spatial and temporal interactions among benthic crustaceans and one fish species in the Bay of Biscay. Mar. Biol. 151, 2207–2215. doi: 10.1007/s00227-007-0655-7
Tunnicliffe, V., Garrett, J. F., and Johnson, H. P. (1990). Physical and biological factors affecting the behaviour and mortality of hydrothermal vent tubeworms (vestimentiferans). Deep Sea Res. A 37, 103–125. doi: 10.1016/0198-0149(90)90031-P
Tunnicliffe, V., Juniper, S. K., and Sibuet, M. (2003). “Reducing environments of the deep-sea floor,” in Ecosystems of the World, vol. 28 Ecosystems of the Deep Oceans, ed. P. A. Tyler (Amsterdam: The Netherlands: Elsevier Science B.V), 81–110.
Vacquié-Garcia, J., Royer, F., Dragon, A. C., Viviant, M., Bailleul, F., and Guinet, C. (2012). Foraging in the darkness of the Southern Ocean: influence of bioluminescence on a deep diving predator. PLoS One 7:e43565. doi: 10.1371/journal.pone.0043565
Van Engeland, T., Godø, O. R., Johnsen, E., Duineveld, G. C. A., and Van Oevelen, D. (2019). Cabled ocean observatory data reveal food supply mechanisms to a cold-water coral reef. Prog. Oceanogr. 162, 51–64. doi: 10.1016/j.pocean.2019.01.007
Van Haren, H., de Jong, M., and Kooijman, P. (2015). Yearlong moored bioluminescence and current data at KM3NeT neutrino telescope sites in the deep Ionian Sea. Astropart. Phys. 67, 1–7. doi: 10.1016/j.astropartphys.2015.01.005
Vereshchaka, A. L., Lunina, A. A., and Sutton, T. (2019). Assessing deep-pelagic shrimp biomass to 3000 m in the Atlantic Ocean and ramifications of upscaled global biomass. Sci Rep. 9, 5946. doi: 10.1038/s41598-019-42472-8
Vic, C., Naveira Garabato, A. C., Green, J. A. M., Waterhouse, A. F., Zhao, Z., Melet, A., et al. (2019). Deep-ocean mixing driven by small-scale internal tides. Nat. Commun. 10:2099. doi: 10.1038/s41467-019-10149-5
Wagner, H. J., Kemp, K., Mattheus, U., and Priede, I. G. (2007). Rhythms at the bottom of the deep sea: cyclic current flow changes and melatonin patterns in two species of demersal fish. Deep Sea Res. I 54, 1944–1956. doi: 10.1016/j.dsr.2007.08.005
Webb, T. J., Vanden Berghe, E., and O’Dor, R. (2010). Biodiversity’s big wet secret: the global distribution of marine biological records reveals chronic under-exploration of the deep pelagic ocean. PLoS One 5:e10223. doi: 10.1371/journal.pone.0010223
Whitney, F. A., Freeland, H. J., and Robert, M. (2007). Persistently declining oxygen levels in the interior waters of the eastern subarctic Pacific. Prog. Oceanogr. 75, 179–199. doi: 10.1016/j.pocean.2007.08.007
Widder, E. (2010). Bioluminescence in the ocean: origins of biological, chemical and ecological diversity. Science 328, 704–708. doi: 10.1126/science.1174269
Widder, E., Johnsen, S., Bernstein, S., Case, J. F., and Neilson, D. J. (1999). Thin layers of bioluminescent copepods found at density discontinuities in the water column. Mar. Biol. 134, 429–437. doi: 10.1007/s002270050559
Wright, G., Gjerde, K., Finkelstein, A., and Currie, D. (2020). Fishing in the Twilight Zone: Illuminating Governance Challenges at the Next Fisheries Frontier. Paris: Institute for Sustainable Development and International Relations (IDDRI). Study N°06/20.
Zhang, X., Jin, L., Chen, C., Guan, D., and Li, M. (2011). Interannual and interdecadal variations in the North Atlantic Oscillation spatial shift. Chin. Sci. Bull. 56, 2621–2627. doi: 10.1007/s11434-011-4607-8
Zhang, Y., Liu, Z., Zhao, Y., Li, J., and Liang, X. (2015). Effect of surface mesoscale eddies on deep-sea currents and mixing in the northeastern South China Sea. Deep-Sea Res. II 122, 6–14. doi: 10.1016/j.dsr2.2015.07.007
Keywords: bioluminescence, deep scattering layer, diel vertical migrations, activity rhythms, monitoring technologies, neutrino telescopes
Citation: Chatzievangelou D, Bahamon N, Martini S, del Rio J, Riccobene G, Tangherlini M, Danovaro R, De Leo FC, Pirenne B and Aguzzi J (2021) Integrating Diel Vertical Migrations of Bioluminescent Deep Scattering Layers Into Monitoring Programs. Front. Mar. Sci. 8:661809. doi: 10.3389/fmars.2021.661809
Received: 31 January 2021; Accepted: 03 May 2021;
Published: 28 May 2021.
Edited by:
Pedro A. Ribeiro, University of Bergen, NorwayReviewed by:
Ellen Pape, Ghent University, BelgiumDoug Bartlett, University of California, San Diego, United States
Copyright © 2021 Chatzievangelou, Bahamon, Martini, del Rio, Riccobene, Tangherlini, Danovaro, De Leo, Pirenne and Aguzzi. This is an open-access article distributed under the terms of the Creative Commons Attribution License (CC BY). The use, distribution or reproduction in other forums is permitted, provided the original author(s) and the copyright owner(s) are credited and that the original publication in this journal is cited, in accordance with accepted academic practice. No use, distribution or reproduction is permitted which does not comply with these terms.
*Correspondence: Damianos Chatzievangelou, damchatzi@gmail.com; Jacopo Aguzzi, jaguzzi@icm.csic.es