Honokiol decreases alpha-synuclein mRNA levels and reveals novel targets for modulating alpha-synuclein expression
- 1Department of Neuroscience, Mayo Clinic, Jackson ville, FL, United States
- 2Mayo Clinic Graduate School of Biomedical Sciences, Mayo Clinic College of Medicine, Rochester, MN, United States
- 3Feinberg School of Medicine, Northwestern University, Chicago, IL, United States
- 4Department of Neurosurgery, Mayo Clinic, Jacksonville, FL, United States
Background: Intracytoplasmic inclusions comprised of aggregated alpha-synuclein (αsyn) represent a key histopathological feature of neurological disorders collectively termed “synucleinopathies,” which includes Parkinson’s disease (PD). Mutations and multiplications in the SNCA gene encoding αsyn cause familial forms of PD and a large body of evidence indicate a correlation between αsyn accumulation and disease. Decreasing αsyn expression is recognized as a valid target for PD therapeutics, with down-regulation of SNCA expression potentially attenuating downstream cascades of pathologic events. Here, we evaluated if Honokiol (HKL), a polyphenolic compound derived from magnolia tree bark with demonstrated neuroprotective properties, can modulate αsyn levels in multiple experimental models.
Methods: Human neuroglioma cells stably overexpressing αsyn, mouse primary neurons, and human iPSC-derived neurons were exposed to HKL and αsyn protein and SNCA messenger RNA levels were assessed. The effect of HKL on rotenone-induced overexpression of αsyn levels was further assessed and transcriptional profiling of mouse cortical neurons treated with HKL was performed to identify potential targets of HKL.
Results: We demonstrate that HKL can successfully reduce αsyn protein levels and SNCA expression in multiple in vitro models of PD with our data supporting a mechanism whereby HKL acts by post-transcriptional modulation of SNCA rather than modulating αsyn protein degradation. Transcriptional profiling of mouse cortical neurons treated with HKL identifies several differentially expressed genes (DEG) as potential targets to modulate SNCA expression.
Conclusion: This study supports a HKL-mediated downregulation of SNCA as a viable strategy to modify disease progression in PD and other synucleinopathies. HKL has potential as a powerful tool for investigating SNCA gene modulation and its downstream effects.
1. Introduction
Alpha-synuclein (αsyn) accumulation is a key feature in the pathogenesis of Parkinson’s disease (PD) and related synucleinopathies (Spillantini et al., 1997). These diseases are characterized by the misfolding and aggregation of αsyn protein that can propagate between cells in the brain and accumulate as Lewy bodies (LB) and Lewy neurites (LN) in susceptible cellular populations (Luk et al., 2012). Expression of αsyn is a strong disease modifier as individuals with a triplication of the SNCA gene locus develop aggressive forms of PD with dementia (Polymeropoulos et al., 1997, Singleton et al., 2003, Houlden and Singleton, 2012). Experimentally, the expression level of αsyn is an important determinant of the rate of fibrillization and neurotoxicity (Rockenstein et al., 2014). Additionally, knocking out αsyn in mice (Dauer et al., 2002) or knocking down αsyn in differentiated human dopaminergic cells increases resistance to the mitochondrial toxin MPP + (Fountaine and Wade-Martins, 2007). Disease-modifying therapies for PD remain a major unmet medical need and reducing αsyn levels is a promising therapeutic target (Junn et al., 2009; Mandler et al., 2015; Schneeberger et al., 2016; Valera et al., 2016; Kallab et al., 2018). Downregulation of αsyn via the use of passive or active immunization (Helmschrodt et al., 2017; Kantor et al., 2018; Brys et al., 2019; Savitt and Jankovic, 2019; Uehara et al., 2019), antisense oligonucleotides strategy (Junn et al., 2009; Dehay et al., 2016; Vaikath et al., 2019), and viral vector technology (Menon et al., 2021) has demonstrated beneficial effects and could attenuate downstream cascades of pathologic events. However, neurotoxicity associated with robust reduction of SNCA mRNA levels was reported in studies that utilized RNAi tools to directly target SNCA transcripts and immunization against αsyn still requires larger efficacy trials. Therefore, further studies are necessary to develop a strategy that safely and successfully down-regulates αsyn.
Phytochemical compounds can contribute to the brain’s chemical balance and current evidence supports the applicability of natural compounds to treat neurodegenerative disorders (Perez-Hernandez et al., 2016; Sharifi-Rad et al., 2020). The medicinal properties of plants are mostly attributed to their secondary phytochemical metabolites that have a wide spectrum of pharmacological activities, including but not limited to, antioxidant, anti-tumor, anti-inflammatory, and neuroprotective properties (Kumar and Khanum, 2012; Forni et al., 2019). Honokiol (HKL) is a polyphenolic compound derived from the bark of magnolia plant that has demonstrated favorable effects in experimental models of cancer (Ong et al., 2019), Alzheimer’s disease (Wang et al., 2018) (Ramesh et al., 2018), and PD (Chen et al., 2018a). Additionally, oral administration of HKL attenuated age-related cognitive impairment and neuronal injury in senescence accelerated mice (Matsui et al., 2009). Importantly, intraperitoneal administration of HKL produced a desirable bioavailability (Wang et al., 2011) with considerable blood brain barrier penetration (Lin et al., 2012).
A previous study demonstrated that chronic HKL treatment prevented dopaminergic neuronal loss and motor impairments in a hemi-parkinsonian mouse model (Chen et al., 2018a). Here, we use multiple cellular models to further characterize potential benefits of HKL in PD. Additionally, we demonstrate that HKL can modulate SNCA expression levels and interrogate the molecular mechanism(s) whereby HKL has its effect on SNCA.
2. Materials and methods
2.1. Wt-αsyn H4 cell culture
A stable H4 neuroglioma cell line expressing human wt-αsyn was generated and previously described (Moussaud et al., 2015). Cells were maintained at 37°C in a 95% air/5% CO2 humidified incubator in Opti-MEM supplemented with 10% FBS, 200 μg/mL G418, and 200 μg/mL Hygromycin. Tetracycline (1 μg/mL, Sigma, #T7660-5G) was added to culture media to block the expression of αsyn in the transgene cells.
2.2. Cortical primary neurons preparation
Pregnant adult CD-1 mice were ordered from Jackson Laboratory (Bar Harbor, ME). Cell culture dishes were freshly prepared for each litter and were coated with poly-D-lysine (PDL) diluted in DPBS at a final concentration of 0.1 mg/mL. All media were made fresh for each litter and used within 1–2 weeks. Dissection buffer was prepared containing 1X HBSS without phenol red, calcium, or magnesium (Gibco, #14185-052) and HEPES (Gibco, #15630-106) at final concentration of 10 mM. Primary culture FBS medium comprised NeuroBasal Medium without L-Glutamine (Gibco, #21103-049), 10% FBS (Gibco, #10437-028), 1% GlutaMAX (Gibco, #35050-061), and 1% Penstrep (Gibco, #15140-122). Neuronal media comprised NeuroBasal medium without L-Glutamine, 2% B-27 (ThermoFisher, # 17504044), 1% GlutaMAX, and 1% Penstrep.
2.3. HKL and DMSO solutions preparation
A 100 mM stock solution of HKL (MedChem express, #HY-N0003-50MG) was dissolved in 100% DMSO and stored at -30o C. For treatment of cells, HKL was further diluted in the appropriate cell culture media at a final concentration of 10 μM. DMSO controls were similarly prepared with a final concentration of 0.01% DMSO. Wt-αsyn and primary cortical neurons were treated with 0.01% DMSO or 10 μM HKL for 72 h before being processed.
2.4. Induced pluripotent stem cell (IPSC)-derived neurons treatment with HKL
Lymphoblast cell lines were acquired from the Coriell Institute for Medical Research, Line # GM15010, female origin (New Jersey, USA) from a patient carrying a triplication in the SNCA gene, reprogrammed into induced pluripotent stem cells (iPSCs) called line ‘3x-1’, and characterized previously (Stojkovska et al., 2022). iPSCs were cultured on Matrigel (Corning, #354277) coated plates and maintained in mTESR1 media. Differentiation into midbrain dopaminergic neurons occurred using previously established protocols (Kriks et al., 2011). Briefly, iPSC lines were accutased (Corning, # 25058CI) and seeded onto Matrigel (Corning, #354277) coated plates, allowed to grow to confluency, then treated with dual SMAD inhibitors followed by a cocktail of growth factors (Cuddy et al., 2019). After differentiation, the neurons were cultured in neurobasal medium (ThermoFisher, # 21103049) with NeuroCult SM1 Neuronal Supplement (Stem cell technologies, #5711) and 1% glutamine and penicillin/streptomycin. Patient derived SNCA triplication IPSC-neurons were used to evaluate the effects of HKL in a human relevant model of synucleinopathy. Neurons were matured for 60 days and subsequently treated with 10 μM HKL or 0.01% DMSO for 72 h. Cells were pelleted, snap frozen, and shipped to Mayo Clinic-Jacksonville for αsyn and SNCA mRNA quantitation.
2.5. Western blotting analysis
To prepare whole cell lysates, cells were washed twice with ice-cold PBS and total proteins were isolated by incubating the cells on ice in radio-immunoprecipitation assay (RIPA) lysis buffer (50 mM Tris–HCl, pH 7.4, 150 mM NaCl, 1 mM EDTA, 1 mM EGTA, 1.2% Triton X-100, 0.5% sodium deoxycholate, and 0.1% SDS) containing 1 mM phenylmethylsulfonyl fluoride (PMSF), protease inhibitor cocktail, and phosphatase inhibitor cocktail. Collected cells were sonicated on ice and centrifuged at 10,000 × g for 10 min at 4°C. The protein concentration was determined with Bradford reagent (Thermo Fisher, #23225). 5–10 μg of total proteins were separated on bis-tris polyacrylamide gradient gels (NuPAGE Novex 4-12% Bis-Tris Gel, Life tech, #NW04120BOX) or TGX stain-free gels (BioRad, #4568126) and transferred to PVDF membranes. Membranes were then blocked for 1 h at room temperature (RT) in TBS-T (500 mM NaCl, 20 mM Tris, 0.1% Tween 20, pH 7.4) supplemented with 5% non-fat dried milk. Subsequently membranes were incubated overnight at 4°C with primary antibodies (see Supplementary Table 1 for list of antibodies) followed by 1 h at RT with HRP-conjugated secondary antibodies. Proteins were detected using an enhanced chemiluminescent detection system (ECL, EMD Millipore, #WBKLS0500) and the BioRad ChemiDoc MP (#12003153) imaging system. Blots were quantified using ImageJ and Image lab software (BioRad, #17006130) and normalized to the appropriate loading control such as Vinculin (Sigma, #V9131), GAPDH (Abgent, #AP7873a), Actin (Sigma, #A5060), or total protein.
2.6. Cell toxicity, viability, and proliferation
Cell toxicity was assessed using the Toxilight bioassay kit (Lonza, #LT17-217) in both primary cortical neurons and in wt-αsyn cells to determine the viability of cells after treatment with HKL. In both cases, cells were treated with 0.01% DMSO or 10 μM HKL for 72 h. The culture plates were removed from the 37°C incubator and left at room temperature for 5 min. 20 μl of conditioned media was transferred from each well to a 96-well luminescence compatible plate. Fresh adenylate kinase (AK) detection reagent was used for each experiment, in which 100μl was added to each conditioned media containing well and incubated at room temperature for 5 min. Luminescence was then read for 1 second in a microplate reader (EnVision, PerkinElmer).
Cell proliferation was determined using WST-1 (abcam, #ab155902) assay according to manufacturer instructions. Briefly, WT-αsyn cells were plated in a 96-well plate with 10,000 cells per well in 100μl of media containing tetracycline. The following day, tetracycline was removed, and the cells were treated with either 0.01% DMSO or 10 μM HKL in 100μl of media. After 24, 48, and 72 h, 10 μl/well of WST-1 reagent was added to each well and the plate was incubated at 37°C for 4 h. WST-1 absorbance was read at 450 nm on an EnVision microplate reader (EnVision, PerkinElmer). Three biological replicates of the cell proliferation assay were performed each with three technical replicates for each group in each set.
2.7. Degradation assays
Wt-αsyn cells were grown in the absence of tetracycline for 24 h. Then, tetracycline was added to suppress further expression of αsyn, and cells were treated with 10 μM HKL or 0.01% DMSO. Cells were then harvested at 0, 6, 12, 24, 48, and 72 h for western blot and qPCR analysis to evaluate rate of αsyn and SNCA mRNA degradation.
2.8. mRNA extraction and qPCR
Total RNA was extracted from cells using TRIzol Reagent (Ambion Life Technology, #15596018) followed by DNase RNA cleanup using RNeasy (Qiagen, #74106). The quantity and quality of RNA samples were determined by the Agilent 2100 Bioanalyzer using an Agilent RNA 6000 Nano Chip. Complementary DNA (cDNA) synthesized with Applied Biosystems High-Capacity cDNA Archive Kit was used as a template for relative quantitative PCR using ABI TaqMan chemistry (Applied Biosystems, #4368814). mRNA expression was quantified using Hs00240906_m1 (human SNCA), Mm01188700_m1(mouse Snca), Mm00497442 _m1 (txnl1, Thioredoxin-Related Protein 1), and Hs02800695_m1(HPRT1, Hypoxanthine-guanine phosphoribosyltransferase) probes (see Supplementary Table 2 for complete list of probes). Each sample was run in quadruplet replicates on the QuantStudio 7 Real-Time PCR System (Thermo Fisher) and quantification was done using the 2–ΔΔCT method.
2.9. RNAscope/immunocytochemistry (ICC)
RNAscope is a variation of FISH (fluorescent in situ hybridization) used to visualize RNA transcripts within cells. Kits and probes were purchased from ACD (Fluorescent multiplex detection reagent kit, #320851 and SNCA, #571241) and used according to manufacturer’s instructions. Briefly, cells were fixed with 4% PFA, SNCA mRNA was amplified, and the secondary fluorescent detection probe (Thermo Fisher, Alexa Fluor 488 #A11000) was added. Cells then underwent ICC to examine αsyn protein levels. Cells were permeabilized using 0.1% Triton-X in 1X PBS and incubated at RT. Following additional washes, bovine serum albumin (BSA) was used to block non-specific antigens. Primary antibody, 4B12 (αsyn, # 807802, 1:1,000) was diluted in blocking buffer and incubated at RT. Secondary fluorescent antibody Alexa Fluor 568 (Thermo Fisher, # A11004, 1:1,000), were incubated after washes in the dark. Hoechst staining (#H3570, 1:5,000) was completed prior to imaging and quantification on a Perkin Elmer Operetta CLS High Content Imager (Johns Creek, GA).
2.10. Honokiol derivatives
Magnolol (#M0125) and 4-0-Methylhonokiol (#M184770) were ordered from LKT Labs (St. Paul, MN). Derivatives arrived in powered form and were prepared in the same manner as HKL (i.e., dissolved in 100% DMSO and diluted to 10 μM in the appropriate culture medium).
2.11. RNA sequencing
The mRNA samples were sequenced by the Mayo Clinic Genome Analysis Core (Rochester, MN) using Illumina HiSeq 4000 (San Diego, CA). Reads were mapped to the mouse genome mm10. The raw gene read counts, along with sequencing quality control, were generated using the Mayo Clinic RNA sequencing (RNA-seq) analytic pipeline: MAP-RSeq version 3.0.1. Conditional quantile normalization (CQN) was performed on raw gene counts to remove biases created by GC content and technical variation, to adjust for gene length and library size differences, and to obtain similar quantile-by-quantile distributions of gene expression across samples. Based on the bimodal distribution of the CQN-normalized and log2-transformed reads per kilobase per million (RPKM) gene expression values, genes with average log2 RPKM ≥ 2 in at least one group were considered to have expression above the detection threshold. Using this selection threshold, 19,005 genes were included in downstream analyses.
2.12. Rotenone treatment
Wt-αsyn cells were plated in 6-well plates at 1 × 105 cells/well with tetracycline. The following day, tetracycline was removed and 0.5 μM rotenone (#R8875,Sigma) was added for 2 h at 37°C. After the 2-h incubation, cells were washed, fresh media was added, and wells were treated with 0.01% DMSO or 10 μM HKL for 72 h then harvested for protein and mRNA analysis.
2.13. Statistical analysis
All data were assessed using Graph Pad Prism 9 software (San Diego, CA) and analyzed by one-way ANOVA with Dunnett’s multiple comparisons test or unpaired Student t-test where appropriate. Proliferation rate of wt-αsyn cells was analyzed with repeated measures two-way ANOVA, followed by Dunnett’s multiple comparisons test. Statistical analysis of degradation rate of αsyn and SNCA mRNA was conducted with paired Student t-test. Differences were considered statistically significant when p < 0.05. Results are presented as mean ± standard error of the mean (SEM).
3. Results
3.1. HKL reduces overexpressed αsyn in vitro
Because HKL has previously been reported to inhibit αsyn amyloid fibril formation in a cell-free aggregation assay (Das et al., 2018) we sought to examine the effect of HKL on αsyn in cellular synucleinopathy models given the critical role this protein plays in PD pathogenesis. Tetracycline-regulated H4 neuroglioma cells stably overexpressing human wt-αsyn were treated with escalating doses of HKL for 72 h, harvested, and αsyn protein levels were assessed by Western blot. While doses of HKL in the range 0.625–5 μM did not alter αsyn levels, 10 μM HKL decreased αsyn protein levels by 39% [F (7,16) = 21.84, p < 0.0001] (Figure 1A). Therefore, we chose to use 10 μM HKL for the remainder of the experiments. Additional experiments confirmed that 10 μM HKL consistently reduces αsyn levels by up to 70% [t (14) = 9.18, p < 0.0001] (Figure 1B). Because αsyn expression in the H4 stable cell line is under control of a constitutively active tetracycline regulated promoter, in support of a specific effect on αsyn expression, we confirmed that HKL has no effect on expression of GFP in a similar tetracycline-regulated GFP-expressing H4 stable cell line (Supplementary Figure 1). Importantly, 10 μM HKL treatment did not induce toxicity and did not affect cell proliferation in H4 wt-αsyn overexpressing cells (Supplementary Figures 2A–C). Together, these findings support HKL as an effective and safe compound to modulate αsyn levels in vitro.
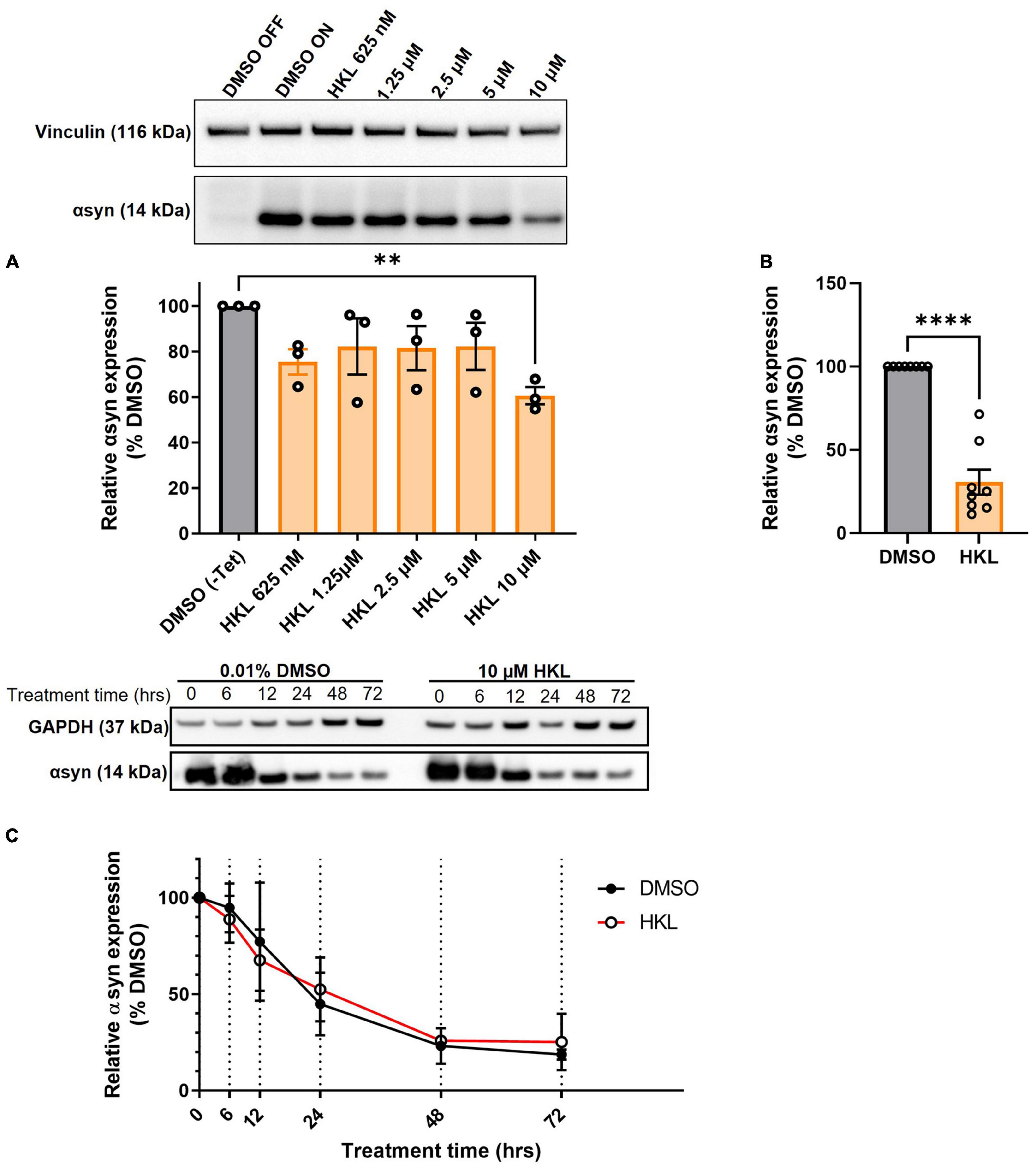
Figure 1. HKL reduces αsyn levels in H4 cells stably overexpressing wt-αsyn. Western blot and quantification of αsyn expression following treatment with different doses of HKL (n = 3 biological replicates/treatment, One-way ANOVA and Dunnet’s post hoc) (A). Confirmation of αsyn expression reduction induced by 10 μM HKL (n = 8 biological replicates/treatment, unpaired Student’s t-test) (B). Western blot and quantification of αsyn levels at 0, 6, 12, 24, 48, and 72 h of 10 μM HKL treatment (n = 3 biological replicates/time point, paired Student’s t-test) (C). Data are represented as mean ± SEM. **p < 0.01; ****p < 0.0001.
3.2. HKL does not increase αsyn degradation
One mechanism by which αsyn expression may be decreased by HKL is via an increase in the rate of protein degradation. Next, we conducted a degradation assay in wt-αsyn cells to determine if increased degradation was the primary mechanism by which HKL regulated αsyn levels (Figure 1C). Here we took advantage of tetracycline regulation to study the degradation rate of αsyn in the presence of HKL or vehicle (DMSO). Cells overexpressing wt-αsyn were cultured in the absence of tetracycline to allow αsyn expression before being treated with 10 μM HKL. At time zero, tetracycline was added to the media to inhibit additional gene expression and samples were collected at 0, 6,12, 24, 48, and 72 h post treatment. Interestingly, HKL did not change the degradation rate of αsyn [t (5) = 0.068, p = 0.95] compared to vehicle treated cells.
3.3. HKL reduces SNCA transcription in cells overexpressing wt-αsyn
If decreased protein expression in the presence of HKL is not due to an increase in rate of degradation, an alternative explanation could be that regulation is occurring at the level of transcription. To test this, we treated H4 cells overexpressing wt-αsyn with HKL for 72 h and assessed SNCA mRNA levels using quantitative PCR. We observed a significant decrease (51%) in SNCA transcripts levels in cells treated with HKL compared to vehicle [t (14) = 5.35, p < 0.0001] (Figure 2A). This decrease was confirmed using RNAscope to visualize and quantify SNCA transcripts and multiplexed with ICC to evaluate corresponding αsyn protein (Figure 2B). We calculated the total number of nuclei per field (n = 25 fields) and normalized the average SNCA mRNA spots and the average αsyn protein fluorescence intensity to the average number of nuclei for each treatment. Taken together, qPCR, RNAscope, and ICC confirm that HKL treatment significantly decreases SNCA transcript levels by 73% [t (6) = 18.85, p < 0.0001] and αsyn expression by 45% [t (6) = 3.94, p < 0.01] while not affecting expression of αTubulin [t (6) = 2.20, p = 0.07] (Figures 2C–E).
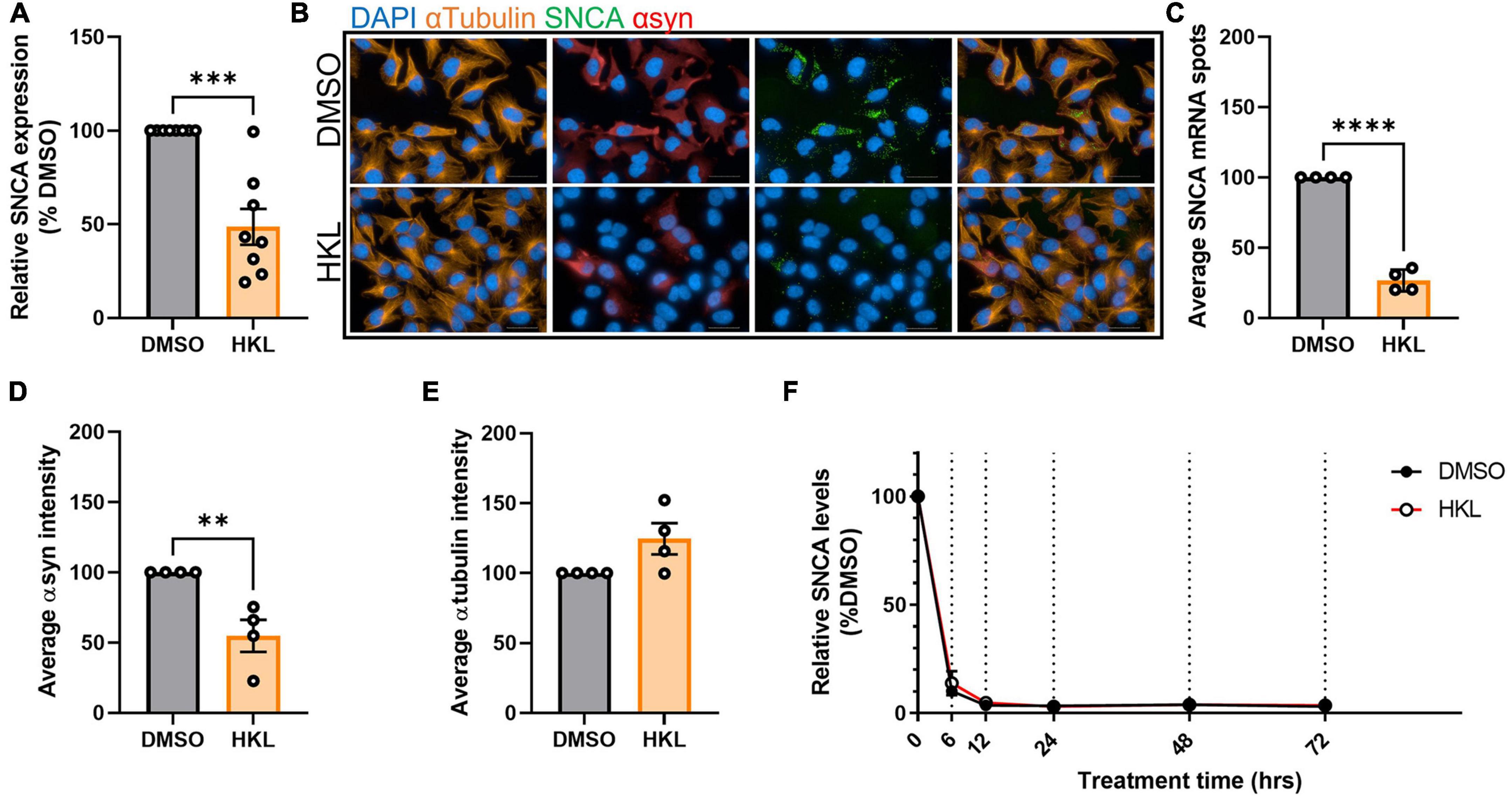
Figure 2. HKL reduces expression of αsyn transcripts. qPCR assay to determine SNCA mRNA levels after 10 μM HKL treatment of H4 wt-αsyn cells (n = 8 biological replicates/treatment) (A). Representative RNAscope images of H4 wt-αsyn cells stained for α-tubulin, SNCA mRNA, and αsyn (B). Four biological replicates with 25 fields/well (2 wells/replicate) were evaluated to determine the effects of HKL and vehicle on SNCA mRNA (C), αsyn (D), and α-tubulin (E). Quantification of SNCA mRNA levels at 0, 6, 12, 24, 48, and 72 h of 10 μM HKL treatment in wt-αsyn cells (n = 3 biological replicates/time point, paired Student’s t-test) (F). Data are analyzed with unpaired Student’s t-test and are represented as mean ± SEM. **p < 0.01, ***p < 0.001, ****p < 0.0001. Scale bars = 200 μm.
3.4. HKL does not affect rate of SNCA mRNA degradation
Our data so far indicate that HKL can modulate αsyn expression and that the modulation is via a transcription related mechanism. The nature of the H4 overexpressing cells (wt-αsyn) excludes the possibility that regulation is at the level of the promoter; thus, we examined whether HKL modulates SNCA levels post-transcriptionally. Here we again took advantage of our tetracycline regulated cell lines to evaluate the rate of mRNA degradation using the same paradigm used previously to examine rate of protein degradation. Interestingly, we determined that HKL does not affect the degradation rate of SNCA mRNA compared to vehicle control (Figure 2F) [t (5) = 1.38, p = 0.22].
3.5. HKL reduces αsyn in mouse primary cortical and but not in iPSC-derived neurons
Because our stable cell lines expressing wt-αsyn are under the control of a constitutively active promoter yet our data support HKL reducing expression by altering levels of transcription, we examined the effect of HKL on endogenous levels of αsyn where expression is controlled by the endogenous promoter. To evaluate the effect of HKL on endogenous αsyn expression, mouse primary cortical neurons were treated at 7 days-in vitro with escalating doses of HKL for 72 h. Interestingly, HKL reduced Snca mRNA levels in mouse primary cortical neurons at a dose as low as 6μM (Figure 3A) [F (5,12) = 8.67, p < 0.01]. To be consistent, however, we continued to use 10 μM HKL in subsequent experiments. Consistent with our previous data, 10 μM HKL treatment resulted in a 44% decrease in αsyn protein expression (Figure 3B) [t (10) = 9.05, p < 0.0001] and a 25% decrease in Snca mRNA levels (Figure 3C), [t (16) = 7.16, p < 0.0001] compared to vehicle (DMSO) treatment.
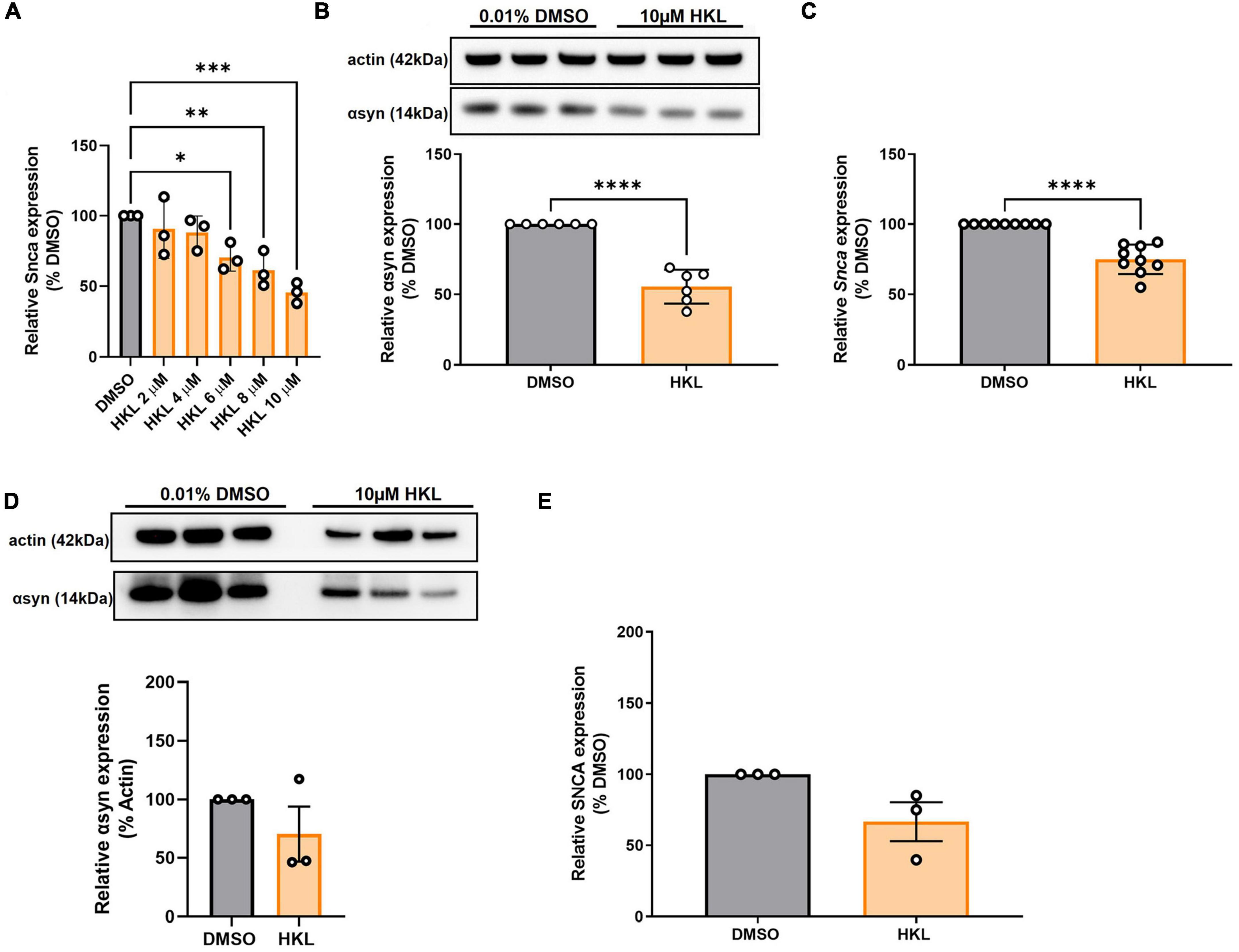
Figure 3. HKL reduces Snca mRNA and αsyn levels in mouse primary cortical neurons but not in human-derived iPSC harboring SNCA triplication. Verification of effective dose of HKL on Snca levels in mouse primary cortical neurons (n = 3 biological replicates/treatment, One-way ANOVA and Dunnet’s post hoc) (A). Confirmation of αsyn expression reduction (n = 3 biological replicates/treatment) (B) and Snca expression reduction (n = 4 biological replicates/treatment) induced by 10 μM HKL (C). Western blot and quantification of αsyn expression following 10 μM HKL treatment on iPSCs (n = 3 biological replicates/treatment) (D). Levels of SNCA mRNA following 10 μM HKL treatment (n = 3 biological replicates/treatment) (E). Data are analyzed with unpaired Student’s t-test and represented as mean ± SEM. *p < 0.05; **p < 0.01, ***p < 0.001, ****p < 0.0001.
To confirm the effect of HKL on endogenous human SNCA we treated human iPSC-derived neurons harboring the SNCA triplication with 10 μM HKL for 72 h. We observed a non-significant 30% reduction in αsyn protein expression induced by HKL (Figure 3D) [t (4) = 1.26, p = 0.28] and a non-significant 33.4% reduction in SNCA mRNA levels (Figure 3E) [t (4) = 2.43, p = 0.07].
3.6. Chemical analogues of HKL are not effective in modulating αsyn
Magnolol is a structural isomer of HKL also extracted from the bark of magnolia, differing only by the position of one hydroxyl group (Figure 4A), and has been reported to have similar biological effects as HKL (Hoi et al., 2010). Hence, we wanted to determine whether magnolol or 4-O-methyl-honokiol (4-0-M-HKL), a HKL-like derivative with good blood brain barrier permeability (Lee et al., 2011), exhibit similar effects on αsyn regulation. Somewhat surprisingly, we found that magnolol and 4-O-M-HKL have no effect on αsyn [F (3, 8) = 7.79, p < 0.01] and SNCA mRNA levels [F (3,8) = 5.91, p < 0.05] (Figures 4B, C). This finding highlights the specificity and robustness of HKL in regulating αsyn expression and SNCA modulation.
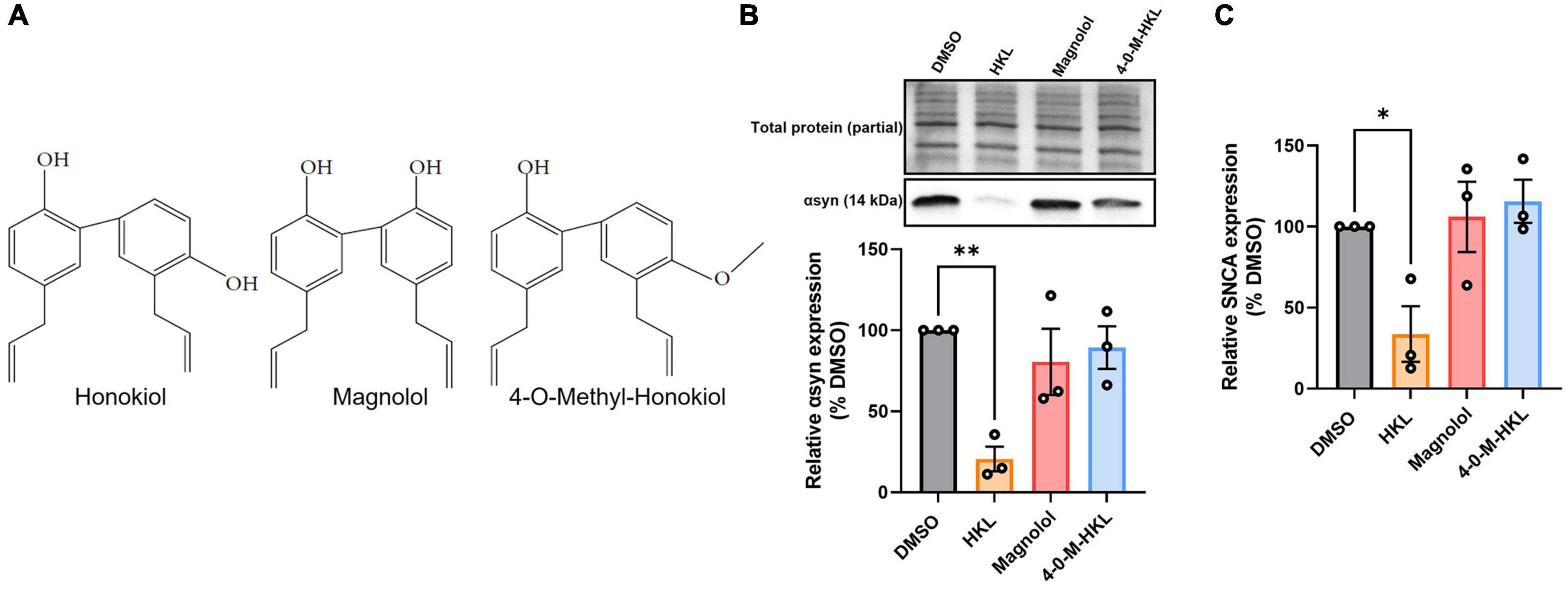
Figure 4. HKL analogues do not modulate expression of αsyn and SNCA mRNA in H4 wt-αsyn cells. Structures of HKL, Magnolol, and 4-O-M-HKL (A) Western blot and quantification of αsyn expression following 72 h treatment with 10 μM HKL, 10 μM Magnolol, and 10 μM 4-0-Methyl-HKL (B). Levels of SNCA mRNA following treatment with HKL and analogues (C). Data are analyzed with one-way ANOVA followed by Dunnet’s post hoc test and are represented as mean ± SEM (n = 3 biological replicates/treatment). *p < 0.05, **p < 0.01.
3.7. HKL differentially regulates gene expression
To further assess specific genetic regulatory targets of HKL we conducted bulk RNA sequencing of mouse primary cortical neurons treated with 10 μM HKL or vehicle (DMSO) for 72 h. As expected, transcriptomic analysis confirmed Snca down-regulation in HKL treated cells and identified numerous differentially expressed gene (DEG) targets between groups (Figure 5). Combining a discovery and replication dataset from 2 mouse litters revealed a total of 293 DEGs. Importantly, among the top 25 DEGs, three major classes of targets were identified, and these encode for proteins involved in myelination (Bcas1), synaptic transmission and cellular communication (Angptl4, Pla2g7), and cell signaling and transmembrane transport (Neat1, Gfap). To further validate these findings, we selected 4 DEGs and validated the effects of HKL on their expression using quantitative RT-PCR. Consistent with our bulk RNA sequencing data Angptl4 and Neat1 were significantly upregulated by HKL and Snca, Cav1, and Kcnq3 were all significantly downregulated by HKL (Supplementary Figure 3). Further investigation and pathway analysis will be required to clarify the specific cellular pathways modulated by HKL that may directly or indirectly lead to the reduction of αsyn levels. These data support HKL as a potential new tool to identify pathways contributing to αsyn pathology and identify potential therapeutic targets.
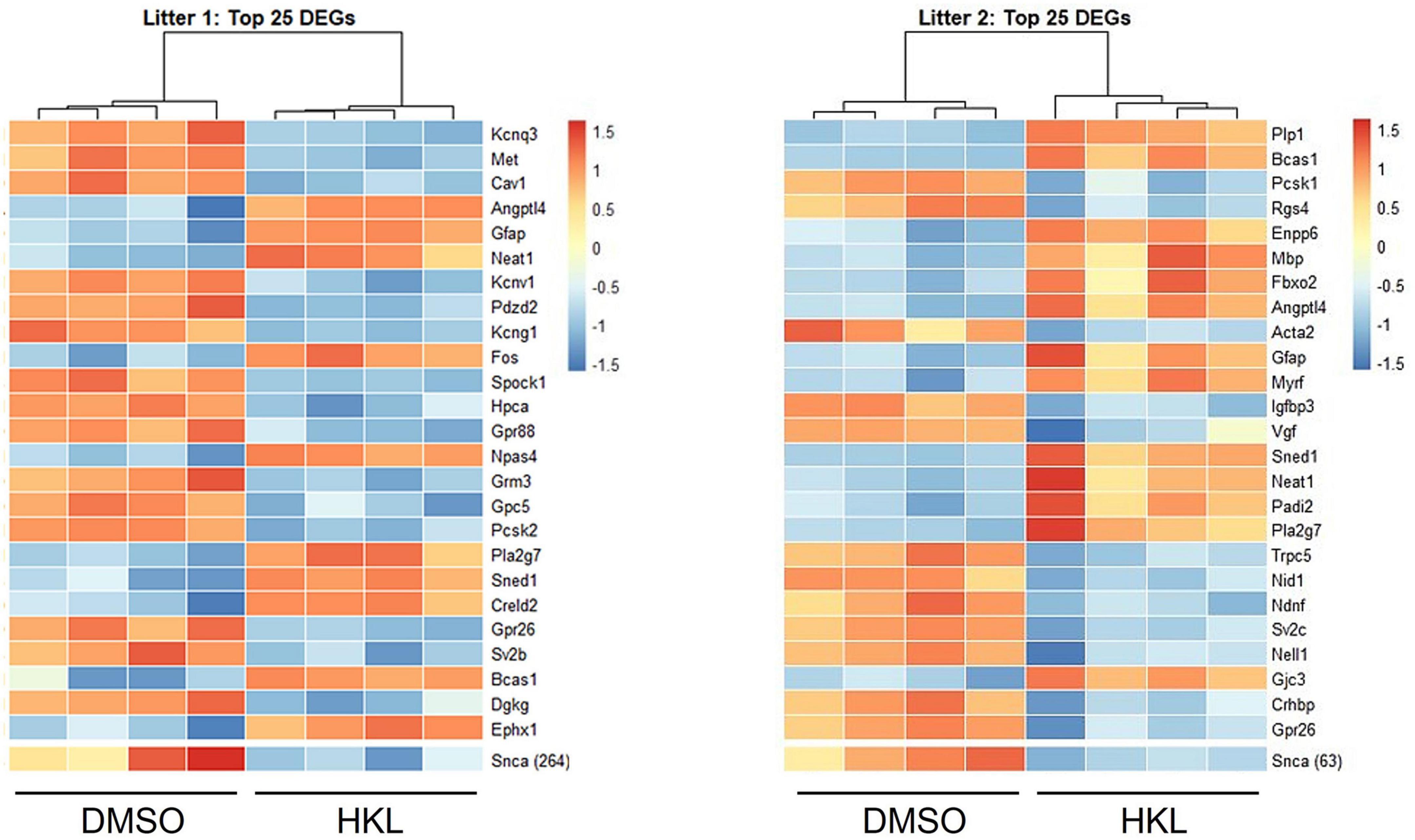
Figure 5. HKL modulates genes associated with myelination, cell signaling and synaptic transmission. Bulk RNA sequencing analysis of 2 litters was performed and revealed 293 differentially expressed genes (DEGs).
3.8. HKL reduces rotenone-induced αsyn expression
Because HKL reduces SNCA/Snca and αsyn levels in multiple cellular models, we next asked if HKL can modulate αsyn levels under pathological conditions. Abundant data supports the fact that exposure to rotenone, a worldwide-used pesticide, is associated with human parkinsonism and in vitro treatment with rotenone has been widely used to model synucleinopathy while in vivo rotenone treatment can induce a parkinsonian-like phenotype with nigrostriatal degeneration (Sanders and Timothy Greenamyre, 2013; De Miranda et al., 2018). When H4 wt-αsyn cells are treated with rotenone we observe a significant increase in αsyn protein levels [F (2,18) = 21.08, p < 0.0001] and SNCA mRNA expression [F (2,6) = 21.57, p < 0.01](Figures 6A, C). Subsequent treatment with 10 μM HKL after rotenone exposure reduced both αsyn protein and SNCA expression levels indicating that HKL can prevent a rotenone-induced cellular response [αsyn: t (12) = 4.02, p < 0.01; SNCA: t (4) = 12.90, p < 0.001] (Figures 6B, D).
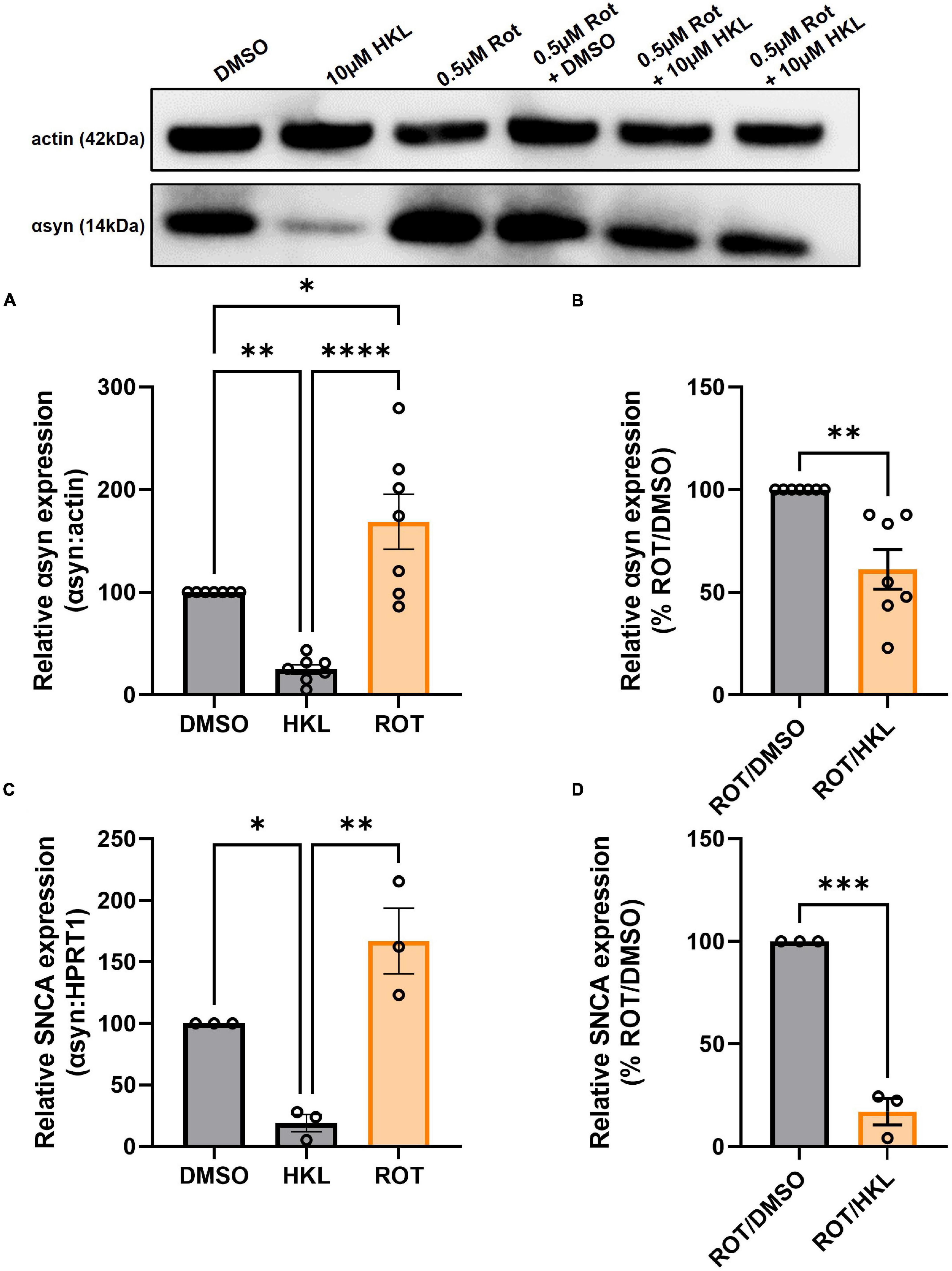
Figure 6. HKL reduces αsyn levels in H4 wt-αsyn cells treated with rotenone. Western blot and quantification of αsyn expression following treatment with 10 μM HKL and 0.5 μM rotenone (n = 7 biological replicates/treatment, One-way ANOVA and Dunnet’s post hoc) (A). Western blot and quantification of rotenone-induced αsyn expression after HKL and DMSO treatment (n = 7 biological replicates/treatment, Student’s t-test) (B). qPCR assay to determine SNCA mRNA levels after 10 μM HKL and 0.5 μM rotenone treatment (n = 3 biological replicates/treatment, One-way ANOVA and Dunnet’s post hoc) (C). qPCR analysis of rotenone-induced SNCA mRNA levels after HKL and DMSO treatment (n = 3 biological replicates/treatment, Student’s t-test) (D). Data are represented as mean ± SEM. *p < 0.05; **p < 0.01; ***p < 0.001; ****p < 0.0001.
4. Discussion
PD is neuropathologically characterized by intracellular inclusions of aggregated αsyn. Multiplications of the SNCA gene locus increases the risk of PD, making αsyn attenuation an important target for drug discovery (Singleton et al., 2003; Olanow and Kordower, 2017). Current therapeutics for PD include medications to promote dopamine production, such as Levodopa, and surgical interventions such as deep brain stimulation (DBS), for advanced stage patients (Groiss et al., 2009; Salat and Tolosa, 2013). These treatments are useful in reducing the symptoms of the disease but are not effective to slow the progression of the disease.
In the present study, we demonstrate that HKL, a natural, brain permeable small molecule, can successfully reduce αsyn expression in multiple in vitro models of PD, which may present a way to slow disease progression. In the human H4 neuroglioma cell line stably overexpressing wt-αsyn under tetracycline regulation, we provide evidence that 10 μM HKL treatment is non-toxic and able to efficiently reduce αsyn and SNCA mRNA levels (Figures 1A, B, 2A–D and Supplementary Figures 2A–C). Similar effects are observed in mouse primary cortical neurons (Figures 3A–C) and in patient derived SNCA iPSC-derived neurons carrying the PD-associated SNCA triplication (Figures 3D, E), although we note that αsyn protein and mRNA level decreases did not quite reach statistical significance in the latter. Our data suggest that the effect of HKL is not mediated by increased rates of protein or mRNA degradation (Figures 1C, 2F). Additionally, we found that the effects on αsyn and SNCA mRNA expression are specific to HKL, as they were not reproduced with structurally-related HKL analogues, including Magnolol, an isomer of HKL (Figure 4). Further, we evaluated the effect of HKL in a pathological environment of αsyn overexpression and showed that HKL reverses rotenone-induced overexpression of αsyn and SNCA mRNA levels (Figure 6). Finally, we took steps towards identifying a mechanism by which HKL may produce these effects by highlighting genes that are differentially regulated in response to HKL treatment (Figure 5 and Supplementary Figure 3).
Previous research has shown that HKL also prevents formation of αsyn aggregates, possibly by stabilizing αsyn native conformation (Das et al., 2018). Additionally, mice with a unilateral 6-OHDA striatal lesion that undergo sub-chronic treatment with HKL demonstrate improvements in motor function, attenuation of nigrostriatal dopaminergic neuronal loss, and reduction in oxidative stress (Chen et al., 2018b). We used H4 cells stably overexpressing αsyn as an in vitro synucleinopathy model (Delenclos et al., 2019) to triage any effects of HKL on αsyn and SNCA mRNA levels. In line with previous results, we observed significant and consistent reduction in αsyn levels (Figures 1B, 2D, 4B, 6A, B) and demonstrated, for the first time, that HKL reduces SNCA gene expression (Figures 2A–C, 4C, 6C, D). Notably, in contrast to previous reports of cell cycle arrest and apoptosis induced by HKL in H4 cells after 48-h treatment (Guo et al., 2015), we did not observe significant toxicity or changes in proliferation rate associated with HKL treatment (Supplementary Figures 2A–C).
Importantly, our primary cortical neurons physiologically expressing αsyn under the mouse Snca promoter provide a more physiologically relevant neuronal environment and allow us to recapitulate the effects of HKL in reducing αsyn protein and mRNA levels (Figures 3A–C). Finally, using iPSC-derived neurons from patients harboring the SNCA gene triplication, we tested the effects of HKL on a physiologically relevant PD model. Our results indicate a non-significant decrease in SNCA mRNA and protein levels (Figures 3D, E), most likely because of the small number of biological replicates included in the current study. Additional studies will need to be conducted to clarify the different transcriptional and post-transcriptional effects on the Snca and SNCA genes. Nonetheless, it is important to note that HKL promotes an overall reduction in αsyn and mRNA levels in different cell models, but the effect size may differ among the models. Therefore, caution is necessary when investigating the mechanisms of in vitro αsyn modulation induced by HKL.
HKL, magnolol, and 4-O-M-HKL are polyphenols with known antioxidant, anti-inflammatory, and anti-tumor effects (Liu et al., 2008; Lee et al., 2009; Shen et al., 2010). Recent evidence even proposes these compounds to have neuroprotective potential (Kumar and Khanum, 2012). Although these compounds are structurally similar and share mechanisms to exert their effects (Woodbury et al., 2013), magnolol and 4-O-M-HKL did not modulate levels of αsyn and SNCA mRNA in our experiments (Figure 4). This finding suggests that HKL has a unique mechanism of action to regulate αsyn.
It has been suggested that HKL modulates the amyloidogenic pathway by activating Sirtuin-3 (SIRT3) exerting antioxidant activity and improving mitochondrial function (Ramesh et al., 2018). The mechanism of HKL in regulating αsyn aggregation has not been clearly elucidated, but recent evidence suggests HKL inhibits fibril formation by directly interacting with lysine-rich region of the N-terminus of the A53T αsyn (Das et al., 2018; Jovcevski et al., 2020). Here, we demonstrate that regulation of αsyn and mRNA levels by HKL do not result from increased rates of transcript and protein degradation (Figures 1C, 2F), and are probably not a direct regulation of transcription, indicating that HKL could be acting to post-transcriptionally modulate SNCA and Snca genes, thus expression of αsyn would be reduced.
Our RNAseq results are in line with this hypothesis by demonstrating that the SNCA gene was downregulated in cultures from two mouse litters subjected to sequencing (Figure 5). Furthermore, we identified other genes that were differentially expressed after HKL treatment. Of interest, Angptl4 is an up-regulated gene that encodes angiopoietin-like 4, a secreted protein that modulates triacylglycerol homeostasis (Koliwad et al., 2012). Indeed, HKL is a partial agonist of peroxisome proliferator-activated receptor-gamma (PPARγ) and was shown to have its neuroprotective effects inhibited by PPARγ antagonists in a hemi-parkinsonian mouse model (Chen et al., 2018b). Considering that PPARγ signaling may influence expression and activity of several genes associated with redox balance, fatty acid oxidation, immune response, and mitochondrial function (Corona et al., 2014), it is possible that HKL indirectly modulates αsyn expression via PPARγ activation. Further studies will be required to confirm or refute this hypothesis. Another potential pathway for αsyn modulation induced by HKL is via the long-non-coding RNA Neat1, an essential structural component of nuclear paraspeckles that has been found increased in the brains and leukocytes of PD patients (Boros et al., 2020). It is possible that Neat1 suppresses the expression of hyper edited Snca transcripts through nuclear retention, and/or inhibition of nuclear-cytoplasm transport (Prasanth et al., 2005; Chen and Carmichael, 2009). Of note, it has been reported that HKL is a modulator of sirtuin3 and other AMPKα-CREB signaling pathways (Ramesh et al., 2018). However, we have not observed significant changes related to Sirtuin3 and AMPKα-CREB signaling-associated genes in our cellular models following HKL treatment (unpublished data).
In summary, we demonstrate that the natural small molecule HKL can modulate levels of αsyn protein and transcript in multiple cell models of synucleinopathies. We also provide initial evidence of mechanisms by which HKL regulates αsyn, which suggests both direct gene regulation and indirect metabolism regulation. Additional studies will need to validate these findings in in vivo PD models and to clarify pathways through which HKL reduces αsyn.
Data availability statement
Original datasets are available in a publicly accessible repository: https://www.synapse.org/#!Synapse:syn51376668.
Author contributions
MD and PJM contributed to the conception and design of the study. SJF, JDB, MJL, DA, ZBK, AHF, IS, DJQ, PP, and NND conducted the experiments, collected, and analyzed the data. SJF wrote the first draft of the manuscript. SLB contributed to data analysis and the first draft of the manuscript. SJF, SLB, PJM, JDB, and NND contributed to the manuscript revision. All authors contributed to the manuscript revision, read, and approved the submitted version.
Funding
Funding was provided by the National Institutes of Health (NS110085 and NS110435), the American Parkinson Disease Association, and the National Institute of Neurological Disorders and Stroke of the National Institutes of Health Award Number RF1NS109157.
Acknowledgments
We would like to thank Christine Perez-Rosa and Morgan Russ for their technical support on this project as well as the Mayo Clinic Genome Analysis Core for performing the RNA sequencing and analysis.
Conflict of interest
The authors declare that the research was conducted in the absence of any commercial or financial relationships that could be construed as a potential conflict of interest.
Publisher’s note
All claims expressed in this article are solely those of the authors and do not necessarily represent those of their affiliated organizations, or those of the publisher, the editors and the reviewers. Any product that may be evaluated in this article, or claim that may be made by its manufacturer, is not guaranteed or endorsed by the publisher.
Supplementary material
The Supplementary Material for this article can be found online at: https://www.frontiersin.org/articles/10.3389/fnagi.2023.1179086/full#supplementary-material
Supplementary Figure 1 | HKL does not affect GFP expression in H4 cells stably overexpressing wt-αsyn. Western blot and quantification of GFP expression following treatment with 10 μM HKL [n = 3 biological replicates/treatment, t(4) = 0.58, p = 0.59] (A). Effects of HKL treatment in eGFP mRNA levels [n = 3 biological replicates/treatment, t (4)1.25, p = 0.28] (B). Data are analyzed with unpaired Student’s t-test and are represented as mean ± SEM.
Supplementary Figure 2 | HKL does not induce toxicity nor affect cell proliferation. The Toxilight assay was performed in H4 wt-αsyn cells after 72 h treatment with 10 μM HKL [n = 5 biological replicates, t (8) = 0.10, p = 0.35] (A). Proliferation WST-1 assay in H4 wt-αsyn cells assessed over 72 h of HKL treatment (n = 3 biological replicates, repeated measures two-way ANOVA, time F (1.13, 9.03) p < 0.05, treatment F (3, 8) = 0.35 p = 0.35, time X treatment F (6, 16) = 0.17 p = 0.98) (B). Toxicity of 72 h treatment with 10 μM HKL on mouse primary cortical neuron was evaluated in the Toxilight assay [n = 3 biological replicates, t (4) = 0.06, p = 0.96] (C). Data are analyzed with unpaired Student’s t-test and are represented as mean ± SEM.
Supplementary Figure 3 | Validation of DEGs in mouse primary cortical neurons. Of the top 25 differentially expressed genes (DEGs) identified with bulk RNA sequencing in mouse primary cortical neurons, the following were validated to confirm the effect of HKL in these cells. Data were analyzed with Student’s t-test and are represented as mean ± S.E.M. ****Cav1 - t (4) = 21.12, p = < 0.0001, **Kcnq3 - t (6) = 4.21, p = 0.0056, ****Angplt4 - t (8) = 8.82, p = < 0.001, *Neat1, t (8) = 2.95, p = 0.0184, ***Snca t (8) = 5.79, p = 0.0004.
References
Boros, F. A., Maszlag-Török, R., Vécsei, L., and Klivényi, P. (2020). Increased level of NEAT1 long non-coding RNA is detectable in peripheral blood cells of patients with Parkinson’s disease. Brain Res. 1730:146672. doi: 10.1016/j.brainres.2020.146672
Brys, M., Fanning, L., Hung, S., Ellenbogen, A., Penner, N., Yang, M., et al. (2019). Randomized phase I clinical trial of anti-α-synuclein antibody BIIB054. Mov. Disord. 34, 1154–1163. doi: 10.1002/mds.27738
Chen, H. H., Chang, P. C., Chen, C., and Chan, M. H. (2018a). Protective and therapeutic activity of honokiol in reversing motor deficits and neuronal degeneration in the mouse model of Parkinson’s disease. Pharmacol. Rep. 70, 668–676. doi: 10.1016/j.pharep.2018.01.003
Chen, H. H., Chang, P. C., Wey, S. P., Chen, P. M., Chen, C., and Chan, M. H. (2018b). Therapeutic effects of honokiol on motor impairment in hemiparkinsonian mice are associated with reversing neurodegeneration and targeting PPARγ regulation. Biomed. Pharmacother. 108, 254–262.
Chen, L. L., and Carmichael, G. G. (2009). Altered nuclear retention of mRNAs containing inverted repeats in human embryonic stem cells: Functional role of a nuclear noncoding RNA. Mol. Cell 35, 467–478. doi: 10.1016/j.molcel.2009.06.027
Corona, J. C., de Souza, S. C., and Duchen, M. R. (2014). PPARγ activation rescues mitochondrial function from inhibition of complex I and loss of PINK1. Exp. Neurol. 253, 16–27.
Cuddy, L. K., Wani, W. Y., Morella, M. L., Pitcairn, C., Tsutsumi, K., Fredriksen, K., et al. (2019). Stress-induced cellular clearance is mediated by the SNARE protein ykt6 and disrupted by α-synuclein. Neuron 104, 869–884. doi: 10.1016/j.neuron.2019.09.001
Das, S., Pukala, T. L., and Smid, S. D. (2018). Exploring the structural diversity in inhibitors of α-synuclein amyloidogenic folding, aggregation, and neurotoxicity. Front. Chem. 6:181. doi: 10.3389/fchem.2018.00181
Dauer, W., Kholodilov, N., Vila, M., Trillat, A. C., Goodchild, R., Larsen, K. E., et al. (2002). Resistance of alpha-synuclein null mice to the parkinsonian neurotoxin MPTP. Proc. Natl. Acad. Sci. U.S.A. 99, 14524–14529. doi: 10.1073/pnas.172514599
De Miranda, B. R., Rocha, E. M., Bai, Q., El Ayadi, A., Hinkle, D., Burton, E. A., et al. (2018). Astrocyte-specific DJ-1 overexpression protects against rotenone-induced neurotoxicity in a rat model of Parkinson’s disease. Neurobiol. Dis. 115, 101–114. doi: 10.1016/j.nbd.2018.04.008
Dehay, B., Vila, M., Bezard, E., Brundin, P., and Kordower, J. H. (2016). Alpha-synuclein propagation: New insights from animal models. Mov. Disord. 31, 161–168.
Delenclos, M., Burgess, J. D., Lamprokostopoulou, A., Outeiro, T. F., Vekrellis, K., and McLean, P. J. (2019). Cellular models of alpha-synuclein toxicity and aggregation. J. Neurochem. 150, 566–576.
Forni, C., Facchiano, F., Bartoli, M., Pieretti, S., Facchiano, A., D’Arcangelo, D., et al. (2019). Beneficial role of phytochemicals on oxidative stress and age-related diseases. Biomed. Res. Int. 2019:8748253.
Fountaine, T. M., and Wade-Martins, R. (2007). RNA interference-mediated knockdown of alpha-synuclein protects human dopaminergic neuroblastoma cells from MPP(+) toxicity and reduces dopamine transport. J. Neurosci. Res. 85, 351–363. doi: 10.1002/jnr.21125
Groiss, S. J., Wojtecki, L., Südmeyer, M., and Schnitzler, A. (2009). Deep brain stimulation in Parkinson’s disease. Ther. Adv. Neurol. Disord. 2, 20–28.
Guo, C., Ma, L., Zhao, Y., Peng, A., Cheng, B., Zhou, Q., et al. (2015). Inhibitory effects of magnolol and honokiol on human calcitonin aggregation. Sci. Rep. 5:13556. doi: 10.1038/srep13556
Helmschrodt, C., Hobel, S., Schoniger, S., Bauer, A., Bonicelli, J., Gringmuth, M., et al. (2017). Polyethylenimine nanoparticle-mediated siRNA delivery to reduce alpha-synuclein expression in a model of Parkinson’s disease. Mol. Ther. Nucleic Acids 9, 57–68. doi: 10.1016/j.omtn.2017.08.013
Hoi, C. P., Ho, Y. P., Baum, L., and Chow, A. H. (2010). Neuroprotective effect of honokiol and magnolol, compounds from Magnolia officinalis, on beta-amyloid-induced toxicity in PC12 cells. Phytother. Res. 24, 1538–1542. doi: 10.1002/ptr.3178
Houlden, H., and Singleton, A. B. (2012). The genetics and neuropathology of Parkinson’s disease. Acta Neuropathol. 124, 325–338.
Jovcevski, B., Das, S., Smid, S., and Pukala, T. L. (2020). Polyphenol honokiol and flavone 2’,3’,4’-trihydroxyflavone differentially interact with α-synuclein at distinct phases of aggregation. ACS Chem. Neurosci. 11, 4469–4477. doi: 10.1021/acschemneuro.0c00654
Junn, E., Lee, K. W., Jeong, B. S., Chan, T. W., Im, J. Y., and Mouradian, M. M. (2009). Repression of alpha-synuclein expression and toxicity by microRNA-7. Proc. Natl. Acad. Sci. U.S.A. 106, 13052–13057.
Kallab, M., Herrera-Vaquero, M., Johannesson, M., Eriksson, F., Sigvardson, J., Poewe, W., et al. (2018). Region-specific effects of immunotherapy with antibodies targeting alpha-synuclein in a transgenic model of synucleinopathy. Front. Neurosci. 12:452. doi: 10.3389/fnins.2018.00452
Kantor, B., Tagliafierro, L., Gu, J., Zamora, M. E., Ilich, E., Grenier, C., et al. (2018). Downregulation of SNCA expression by targeted editing of DNA methylation: A potential strategy for precision therapy in PD. Mol. Ther. 26, 2638–2649. doi: 10.1016/j.ymthe.2018.08.019
Koliwad, S. K., Gray, N. E., and Wang, J.-C. (2012). Angiopoietin-like 4 (Angptl4). Adipocyte 1, 182–187.
Kriks, S., Shim, J. W., Piao, J., Ganat, Y. M., Wakeman, D. R., Xie, Z., et al. (2011). Dopamine neurons derived from human ES cells efficiently engraft in animal models of Parkinson’s disease. Nature 480, 547–551. doi: 10.1038/nature10648
Kumar, G. P., and Khanum, F. (2012). Neuroprotective potential of phytochemicals. Pharmacogn. Rev. 6, 81–90.
Lee, Y. K. I, Choi, S., Ban, J. O., Lee, H. J., Lee, U. S., Han, S. B., et al. (2011). 4-O-methylhonokiol attenuated β-amyloid-induced memory impairment through reduction of oxidative damages via inactivation of p38 MAP kinase. J. Nutr. Biochem. 22, 476–486.
Lee, Y. K., Yuk, D. Y., Kim, T. I., Kim, Y. H., Kim, K. T., Kim, K. H., et al. (2009). Protective effect of the ethanol extract of Magnolia officinalis and 4-O-methylhonokiol on scopolamine-induced memory impairment and the inhibition of acetylcholinesterase activity. J. Nat. Med. 63, 274–282. doi: 10.1007/s11418-009-0330-z
Lin, J. W., Chen, J. T., Hong, C. Y., Lin, Y. L., Wang, K. T., Yao, C. J., et al. (2012). Honokiol traverses the blood-brain barrier and induces apoptosis of neuroblastoma cells via an intrinsic bax-mitochondrion-cytochrome c-caspase protease pathway. Neuro Oncol. 14, 302–314. doi: 10.1093/neuonc/nor217
Liu, H., Zang, C., Emde, A., Planas-Silva, M. D., Rosche, M., Kühnl, A., et al. (2008). Anti-tumor effect of honokiol alone and in combination with other anti-cancer agents in breast cancer. Eur. J. Pharmacol. 591, 43–51. doi: 10.1016/j.ejphar.2008.06.026
Luk, K. C., Kehm, V., Carroll, J., Zhang, B., O’Brien, P., Trojanowski, J. Q., et al. (2012). Pathological alpha-synuclein transmission initiates Parkinson-like neurodegeneration in nontransgenic mice. Science 338, 949–953. doi: 10.1126/science.1227157
Mandler, M., Valera, E., Rockenstein, E., Mante, M., Weninger, H., Patrick, C., et al. (2015). Active immunization against alpha-synuclein ameliorates the degenerative pathology and prevents demyelination in a model of multiple system atrophy. Mol. Neurodegener. 10:10. doi: 10.1186/s13024-015-0008-9
Matsui, N., Takahashi, K., Takeichi, M., Kuroshita, T., Noguchi, K., Yamazaki, K., et al. (2009). Magnolol and honokiol prevent learning and memory impairment and cholinergic deficit in SAMP8 mice. Brain Res. 1305, 108–117. doi: 10.1016/j.brainres.2009.09.107
Menon, S., Kofoed, R. H., Nabbouh, F., Xhima, K., Al-Fahoum, Y., Langman, T., et al. (2021). Viral alpha-synuclein knockdown prevents spreading synucleinopathy. Brain Commun. 3:fcab247. doi: 10.1093/braincomms/fcab247
Moussaud, S., Malany, S., Mehta, A., Vasile, S., Smith, L. H., and McLean, P. J. (2015). Targeting alpha-synuclein oligomers by protein-fragment complementation for drug discovery in synucleinopathies. Expert Opin. Ther. Targets 19, 589–603. doi: 10.1517/14728222.2015.1009448
Olanow, C. W., and Kordower, J. H. (2017). Targeting α-synuclein as a therapy for Parkinson’s disease: The battle begins. Mov. Disord. 32, 203–207. doi: 10.1002/mds.26935
Ong, C. P., Lee, W. L., Tang, Y. Q., and Yap, W. H. (2019). Honokiol: A review of its anticancer potential and mechanisms. Cancers 12:48.
Perez-Hernandez, J., Zaldivar-Machorro, V. J., Villanueva-Porras, D., Vega-Avila, E., and Chavarria, A. (2016). A potential alternative against neurodegenerative diseases: Phytodrugs. Oxid. Med. Cell Longev. 2016:8378613. doi: 10.1155/2016/8378613
Polymeropoulos, M. H., Lavedan, C., Leroy, E., Ide, S. E., Dehejia, A., Dutra, A., et al. (1997). Mutation in the alpha-synuclein gene identified in families with Parkinson’s disease. Science 276, 2045–2047.
Prasanth, K. V., Prasanth, S. G., Xuan, Z., Hearn, S., Freier, S. M., Bennett, C. F., et al. (2005). Regulating gene expression through RNA nuclear retention. Cell 123, 249–263.
Ramesh, S., Govindarajulu, M., Lynd, T., Briggs, G., Adamek, D., Jones, E., et al. (2018). SIRT3 activator honokiol attenuates β-Amyloid by modulating amyloidogenic pathway. PLoS One 13:e0190350. doi: 10.1371/journal.pone.0190350
Rockenstein, E., Nuber, S., Overk, C. R., Ubhi, K., Mante, M., Patrick, C., et al. (2014). Accumulation of oligomer-prone alpha-synuclein exacerbates synaptic and neuronal degeneration in vivo. Brain 137, 1496–1513. doi: 10.1093/brain/awu057
Salat, D., and Tolosa, E. (2013). Levodopa in the treatment of Parkinson’s disease: Current status and new developments. J. Parkinsons Dis. 3, 255–269.
Sanders, L. H., and Timothy Greenamyre, J. (2013). Oxidative damage to macromolecules in human Parkinson disease and the rotenone model. Free Radic. Biol. Med. 62, 111–120. doi: 10.1016/j.freeradbiomed.2013.01.003
Savitt, D., and Jankovic, J. (2019). Targeting alpha-synuclein in Parkinson’s disease: Progress towards the development of disease-modifying therapeutics. Drugs 79, 797–810.
Schneeberger, A., Tierney, L., and Mandler, M. (2016). Active immunization therapies for Parkinson’s disease and multiple system atrophy. Mov. Disord. 31, 214–224.
Sharifi-Rad, M., Lankatillake, C., Dias, D. A., Docea, A. O., Mahomoodally, M. F., Lobine, D., et al. (2020). Impact of natural compounds on neurodegenerative disorders: From preclinical to pharmacotherapeutics. J. Clin. Med. 9:1061.
Shen, J. L., Man, K. M., Huang, P. H., Chen, W. C., Chen, D. C., Cheng, Y. W., et al. (2010). Honokiol and magnolol as multifunctional antioxidative molecules for dermatologic disorders. Molecules 15, 6452–6465. doi: 10.3390/molecules15096452
Singleton, A. B., Farrer, M., Johnson, J., Singleton, A., Hague, S., Kachergus, J., et al. (2003). alpha-Synuclein locus triplication causes Parkinson’s disease. Science 302:841.
Spillantini, M. G., Schmidt, M. L., Lee, V. M., Trojanowski, J. Q., Jakes, R., and Goedert, M. (1997). Alpha-synuclein in Lewy bodies. Nature 388, 839–840.
Stojkovska, I., Wani, W. Y., Zunke, F., Belur, N. R., Pavlenko, E. A., Mwenda, N., et al. (2022). Rescue of α-synuclein aggregation in Parkinson’s patient neurons by synergistic enhancement of ER proteostasis and protein trafficking. Neuron 110, 436–451.e11. doi: 10.1016/j.neuron.2021.10.032
Uehara, T., Choong, C. J., Nakamori, M., Hayakawa, H., Nishiyama, K., Kasahara, Y., et al. (2019). Amido-bridged nucleic acid (AmNA)-modified antisense oligonucleotides targeting alpha-synuclein as a novel therapy for Parkinson’s disease. Sci. Rep. 9:7567. doi: 10.1038/s41598-019-43772-9
Vaikath, N. N., Hmila, I., Gupta, V., Erskine, D., Ingelsson, M., and El-Agnaf, O. M. A. (2019). Antibodies against alpha-synuclein: Tools and therapies. J. Neurochem. 150, 612–625.
Valera, E., Spencer, B., and Masliah, E. (2016). Immunotherapeutic approaches targeting amyloid-beta, alpha-synuclein, and tau for the treatment of neurodegenerative disorders. Neurotherapeutics 13, 179–189.
Wang, D., Dong, X., and Wang, C. (2018). Honokiol ameliorates amyloidosis and neuroinflammation and improves cognitive impairment in Alzheimer’s disease transgenic mice. J. Pharmacol. Exp. Ther. 366, 470–478. doi: 10.1124/jpet.118.248674
Wang, X., Duan, X., Yang, G., Zhang, X., Deng, L., Zheng, H., et al. (2011). Honokiol crosses BBB and BCSFB, and inhibits brain tumor growth in rat 9L intracerebral gliosarcoma model and human U251 xenograft glioma model. PLoS One 6:e18490. doi: 10.1371/journal.pone.0018490
Keywords: alpha-synuclein (αSyn), Parkinson’s disease, SNCA, natural compound, polyphenol, therapeutic target
Citation: Fagen SJ, Burgess JD, Lim MJ, Amerna D, Kaya ZB, Faroqi AH, Perisetla P, DeMeo NN, Stojkovska I, Quiriconi DJ, Mazzulli JR, Delenclos M, Boschen SL and McLean PJ (2023) Honokiol decreases alpha-synuclein mRNA levels and reveals novel targets for modulating alpha-synuclein expression. Front. Aging Neurosci. 15:1179086. doi: 10.3389/fnagi.2023.1179086
Received: 10 March 2023; Accepted: 17 July 2023;
Published: 10 August 2023.
Edited by:
Shigeki Arawaka, Osaka Medical and Pharmaceutical University Faculty of Medicine, JapanReviewed by:
Eftychia Vasili, Biomedical Research Institute (BRI), GreeceKensuke Ikenaka, Osaka University, Japan
Hiroyasu Sato, Yamagata University, Japan
Copyright © 2023 Fagen, Burgess, Lim, Amerna, Kaya, Faroqi, Perisetla, DeMeo, Stojkovska, Quiriconi, Mazzulli, Delenclos, Boschen and McLean. This is an open-access article distributed under the terms of the Creative Commons Attribution License (CC BY). The use, distribution or reproduction in other forums is permitted, provided the original author(s) and the copyright owner(s) are credited and that the original publication in this journal is cited, in accordance with accepted academic practice. No use, distribution or reproduction is permitted which does not comply with these terms.
*Correspondence: Pamela J. McLean, mclean.pamela@mayo.edu; Suelen L. Boschen, souza.suelen@mayo.edu
†These authors have contributed equally to this work and share senior authorship