Enhanced laser-driven backward proton acceleration using micro-wire array targets
- 1State Key Laboratory of High Field Laser Physics and CAS Center for Excellence in Ultra-intense Laser Science, Shanghai Institute of Optics and Fine Mechanics, Chinese Academy of Sciences, Shanghai, China
- 2Center of Materials Science and Optoelectronics Engineering, University of Chinese Academy of Sciences, Beijing, China
- 3Shanghai Institute of Applied Physics, Chinses Academy of Sciences, Shanghai, China
- 4Shanghai Advanced Research Institute, Chinese Academy of Sciences, Shanghai, China
- 5Key Laboratory of Nuclear Physics and Ion-Beam Application (MOE), Institute of Modern Physics, Fudan University, Shanghai, China
- 6Department of Physics, Shanghai Normal University, Shanghai, China
- 7School of Physical Science and Technology, ShanghaiTech University, Shanghai, China
Micro-structured targets can be employed to enhance the coupling of laser energy to the high energy density plasma. Here we report on experimental measurement of enhanced proton beam energy from laser-driven micro-wire array (MWA) targets along the backward direction. An ultra-intense (
Introduction
In the past 2 decades, laser-driven proton acceleration has been developed as a new acceleration method. Due to the high peak flux, short pulse duration and small beam size, laser-driven proton sources are promising in various key applications, such as proton therapy [1], high energy density physics, fast ignition [2] and laboratory astrophysics [3]. Several acceleration mechanisms have been studied to produce high energy protons, including the target normal sheath acceleration [4, 5] (TNSA), shock wave acceleration [6], the radiation pressure acceleration [7] (RPA) etc. While many proposals suggested proton energies could reach up to GeV level, the state-of-art experimental value is around
When an ultrahigh contrast femtosecond laser penetrates deep into the MWA, it creates a large volumetrically heated plasma [24–26], which is the major reason for the high-efficiency generation of x-rays and relativistic electrons. Further, the electrons within the wires are pulled out by the laser field and accelerated via direct laser acceleration (DLA) [28], leading to substantial enhancement in both the population and energies of the produced electron beam [23]. These together benefit acceleration of high energy protons via enhanced sheath field [20]. The laser energy is thus efficiently coupled into energetic protons and therefore can be employed to boost the fusion reaction in the vicinity of high energy density plasma [21, 29]. In this case, the substrates of some MWA targets are usually quite thick to maximize the fusion reaction events [21, 22]. Sheath field at the target rear surface cannot be formed efficiently, thus measuring protons or energetic ions accelerated at the target rear becomes challenging. For this reason, detection of the protons emitted from the front surface turns to be a direct approach to obtain information of interaction regions in micro-structured targets. It has been reported that 13 MeV deuterium ions were measured in the laser beam backward direction by irradiating a deuterated nanowire target with a high contrast strong laser, and the highest proton energy was
Some experiments [30, 31] find an almost symmetric behavior for protons accelerated from rear and front sides with the laser intensity range
In this paper, using a laser with a peak intensity of
Experimental results
The experiment was carried out on a femtosecond petawatt laser system at Shanghai Institute of Optics and Fine Mechanics (SIOM), which delivered laser beams with a central wavelength of
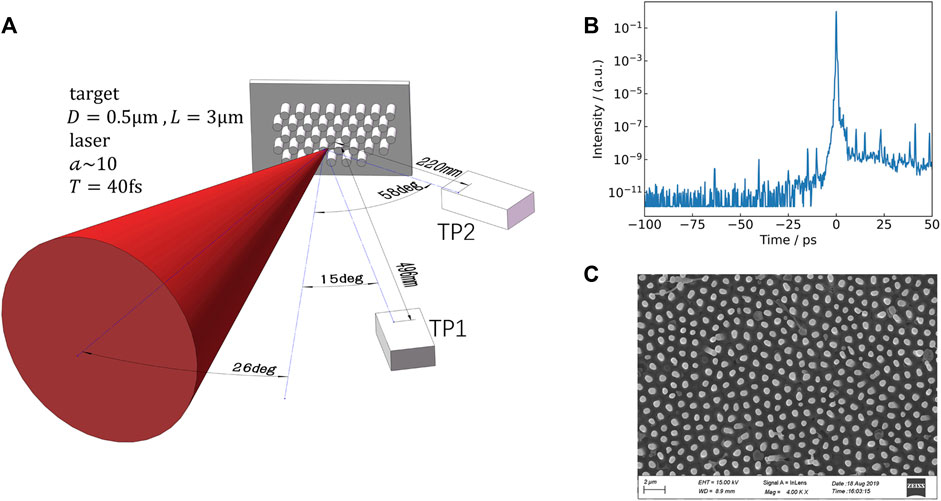
FIGURE 1. (A) Schematic of experimental setup. The laser pulse with
The schematic diagram of the experiment is shown in Figure 1A. The p-polarized laser pulse is incident on the target surface at an angle of
The target is made of a mixture material of polyethylene and boroncarbide powder, with the mass ratio of
The recorded parabolic ion traces on IPs shown in Figure 2A and Figure 2B were analyzed using a MATLAB code applying an existing scaling relationship [36]. Figure 2C shows the proton energy spectra with a wire diameter of
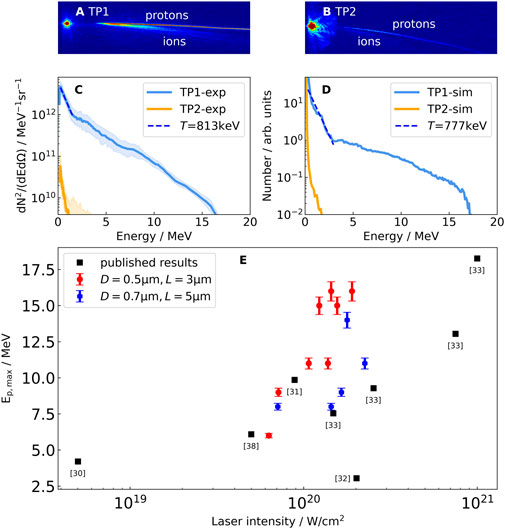
FIGURE 2. (A), (B) The raw IP data of TP1 and TP2 respectively. (C) Experiment energy spectrum of the proton in front of the target with a wire diameter of
The current experiment did not consider the case for flat foils. However, one could refer to previous results with similar laser conditions. In Ref. [30–33, 38], flat foils of thickness >0.1
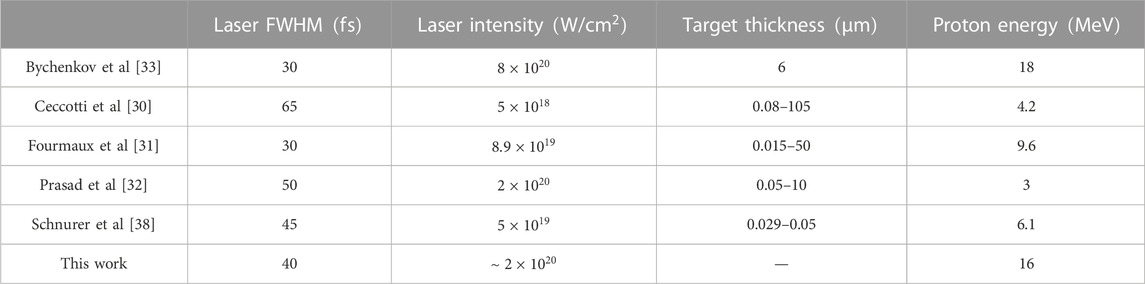
TABLE 1. Laser-driven backward proton acceleration results are shown in comparison with previous experiments.
Simulation results
We use the particle-in-cell (PIC) program SMILEI [39] to interpret the experimental observation. Due to the demanding computation requirement for simulating dense micro-structures, we restrict our simulations to two-dimensional (2D) geometry. Based on the test simulations, we found that the proton acceleration saturates at about 500 fs, corresponding to roughly
The electric field
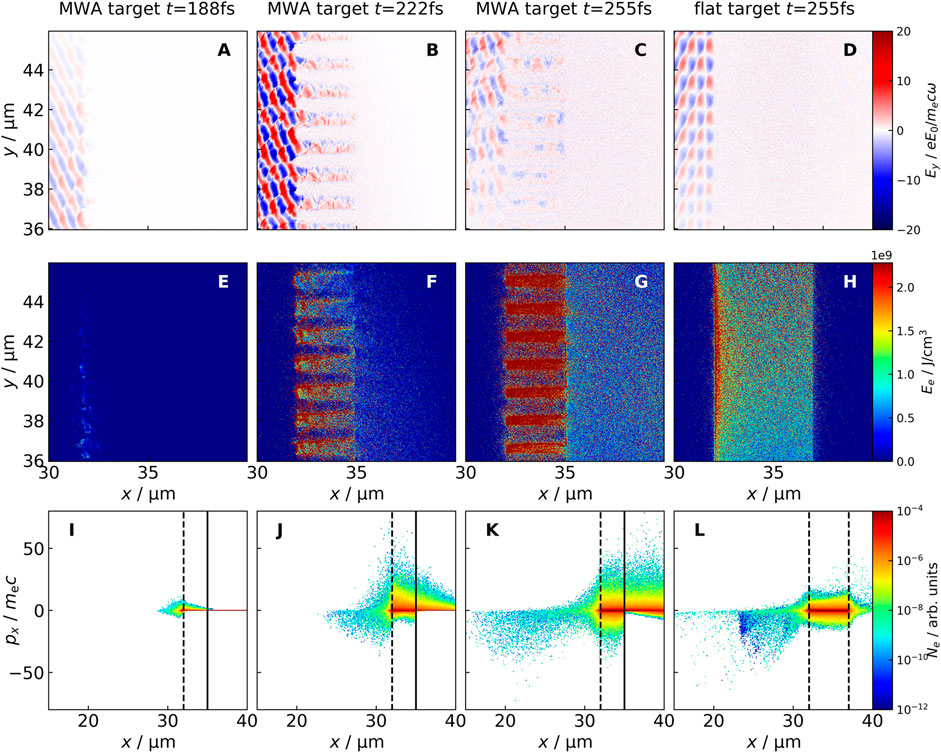
FIGURE 3. 2D PIC simulations results. (A–C) Spatial distribution of the electric field
Figures 4A–D describes the
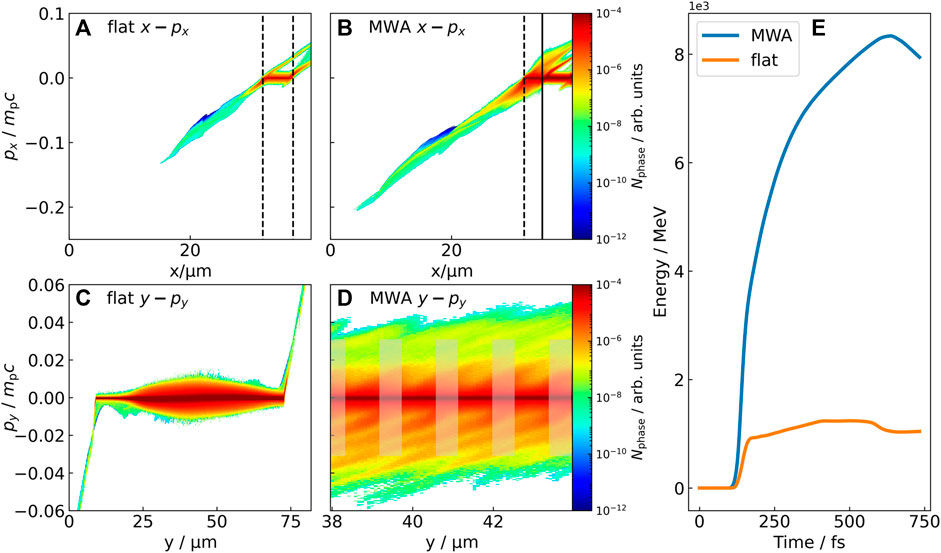
FIGURE 4. The
In Figure 4E we compare the total proton energy obtained from the laser. The energy conversion tends to saturated after
The relative number density distributions of electrons and protons in the energy-angle domain are presented in Figure 5. One can see that there is a gap at
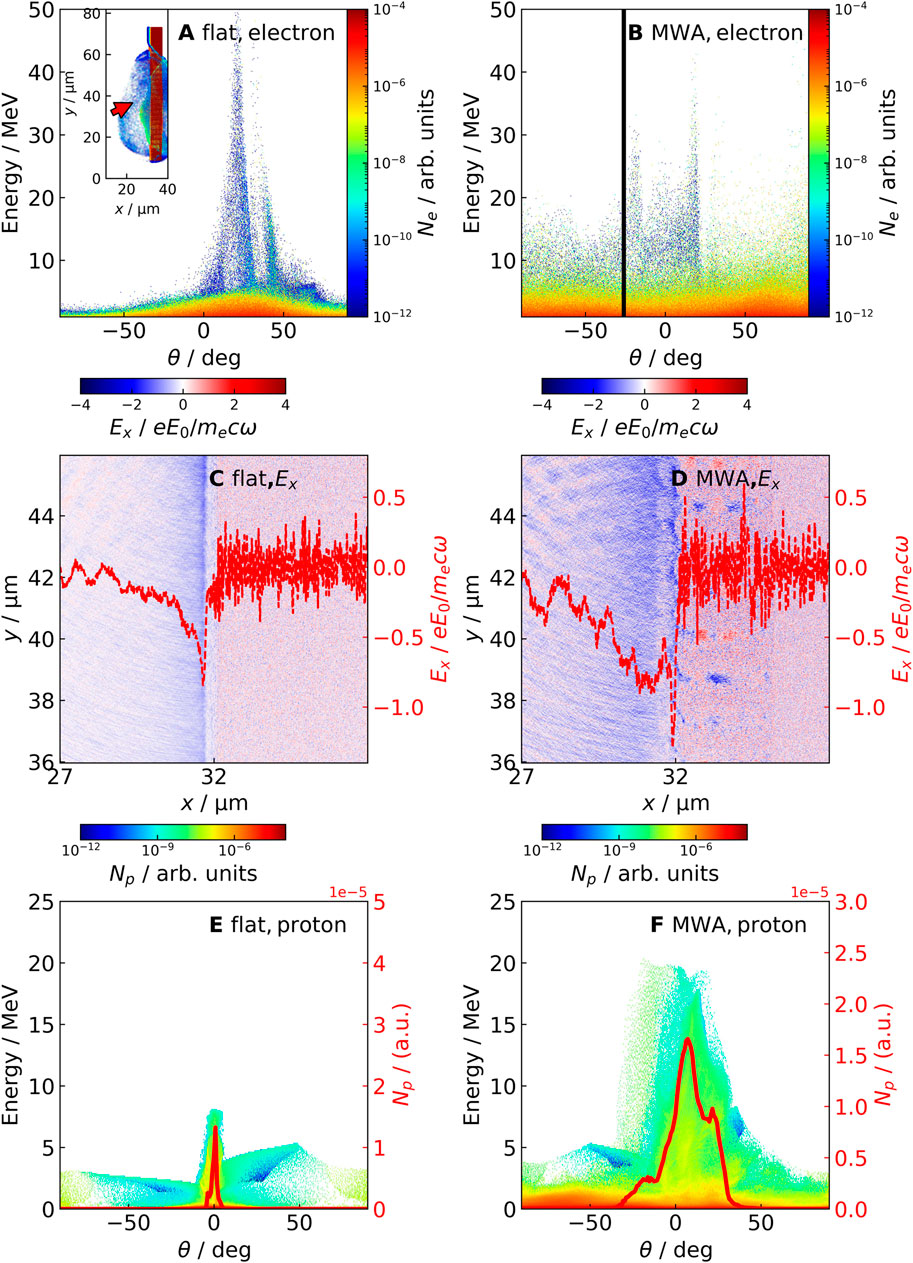
FIGURE 5. The angular-energy distribution of electrons in (A) flat target, (B) micro-wire target at
Discussion
An important feature of the MWA structure is the promising scaling law
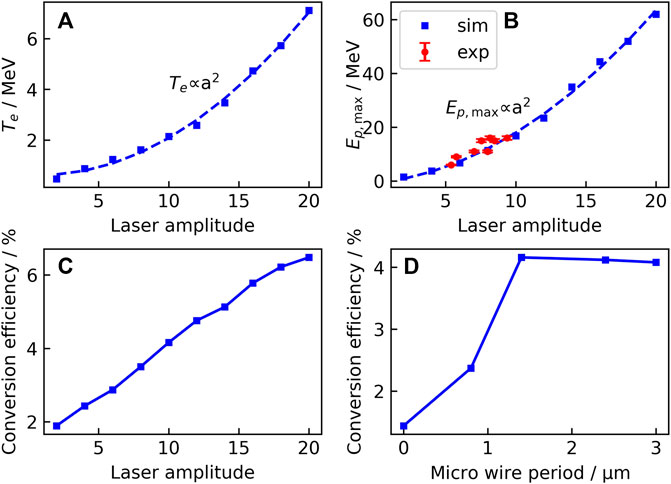
FIGURE 6. (A) The scaling law of laser amplitude and electron temperature. (B) The experiment (red) and simulation (blue) scaling law of laser intensity versus proton maximum energy. The error bars are defined by the uncertainty in maximum proton energy arising from the energy resolution of the Thompson spectrometer. The distance between the wires is
Conclusion
We report on experimental measurement of proton beams with maximum energy of
Data availability statement
The original contributions presented in the study are included in the article/supplementary material, further inquiries can be directed to the corresponding authors.
Author contributions
LF wrote the draft of this paper and joined the experiment. TX proposed the idea, took part in the preparation of the draft and led the experiment. QW and JX joined the experiment. GZ provided the MWA target and led the experiment. PW, CF, ZM, and XD joined the experiment. YM discussed the results. SL joined the experiment. XL, JL, RX, and CW operated the laser device. XL and YL provided a professional laser laboratory. BS discussed the results. LJ proposed the idea, took part in the preparation of the draft and is in charge of the project. RL discussed the results. All authors contributed to the article and approved the submitted version.
Funding
This work was supported by the National Natural Science Foundation of China (Grants Nos. 12175299, 11905278, 11975302, 11935008), the CAS Project for Young Scientists in Basic Research (YSBR060) and Youth Innovation Promotion Association of Chinese Academy of Science (No. 2021242).
Conflict of interest
The authors declare that the research was conducted in the absence of any commercial or financial relationships that could be construed as a potential conflict of interest.
Publisher’s note
All claims expressed in this article are solely those of the authors and do not necessarily represent those of their affiliated organizations, or those of the publisher, the editors and the reviewers. Any product that may be evaluated in this article, or claim that may be made by its manufacturer, is not guaranteed or endorsed by the publisher.
References
1. Bulanov SV, Khoroshkov VS. Feasibility of using laser ion accelerators in proton therapy. Plasma Phys Rep (2002) 28(5):4C53–6. doi:10.1134/1.1478534
2. Roth M, Cowan TE, Key MH, Hatchett SP, Brown C, Fountain W, et al. Fast ignition by intense laser-accelerated proton beams. Phys Rev Lett (2001) 86(3):436–9. Epub 2001/02/15. doi:10.1103/PhysRevLett.86.436
3. Remington BA. High energy density laboratory astrophysics. Plasma Phys Contr F (2005) 47:A191–A203. doi:10.1088/0741-3335/47/5a/014
4. Snavely RA, Key MH, Hatchett SP, Cowan TE, Roth M, Phillips TW, et al. Intense high-energy proton beams from petawatt-laser irradiation of solids. Phys Rev Lett (2000) 85(14):2945–8. Epub 2000/09/27. doi:10.1103/PhysRevLett.85.2945
5. Wilks SC, Langdon AB, Cowan TE, Roth M, Singh M, Hatchett S, et al. Energetic proton generation in ultra-intense laser–solid interactions. Phys Plasmas (2001) 8(2):542–9. doi:10.1063/1.1333697
6. Haberberger D, Tochitsky S, Fiuza F, Gong C, Fonseca RA, Silva LO, et al. Collisionless shocks in laser-produced plasma generate monoenergetic high-energy proton beams. Nat Phys (2011) 8(1):95–9. doi:10.1038/nphys2130
7. Shen B, Xu Z. Transparency of an overdense plasma layer. Phys Rev E Stat Nonlin Soft Matter Phys (2001) 64(5 Pt 2):056406. Epub 2001/12/12. doi:10.1103/PhysRevE.64.056406
8. Wagner F, Deppert O, Brabetz C, Fiala P, Kleinschmidt A, Poth P, et al. Maximum proton energy above 85 Mev from the relativistic interaction of laser pulses with micrometer thick Ch_{2} targets. Phys Rev Lett (2016) 116(20):205002. Epub 2016/06/04. doi:10.1103/PhysRevLett.116.205002
9. Higginson A, Gray RJ, King M, Dance RJ, Williamson SDR, Butler NMH, et al. Near-100 Mev protons via a laser-driven transparency-enhanced hybrid acceleration scheme. Nat Commun (2018) 9(1):724. Epub 2018/02/22. doi:10.1038/s41467-018-03063-9
10. Zigler A, Eisenman S, Botton M, Nahum E, Schleifer E, Baspaly A, et al. Enhanced proton acceleration by an ultrashort laser interaction with structured dynamic plasma targets. Phys Rev Lett (2013) 110(21):215004. ARTN 215004. doi:10.1103/PhysRevLett.110.215004
11. Ceccotti T, Floquet V, Sgattoni A, Bigongiari A, Klimo O, Raynaud M, et al. Evidence of resonant surface-wave excitation in the relativistic regime through measurements of proton acceleration from grating targets. Phys Rev Lett (2013) 111(18):185001. ARTN 185001. doi:10.1103/PhysRevLett.111.185001
12. Ma WJ, Kim IJ, Yu JQ, Choi IW, Singh PK, Lee HW, et al. Laser acceleration of highly energetic carbon ions using a double-layer target composed of slightly underdense plasma and ultrathin foil. Phys Rev Lett (2019) 122(1):014803. ARTN 014803. doi:10.1103/PhysRevLett.122.014803
13. Margarone D, Klimo O, Kim IJ, Prokupek J, Limpouch J, Jeong TM, et al. Laser-driven proton acceleration enhancement by nanostructured foils. Phys Rev Lett (2012) 109(23):234801. ARTN 234801. doi:10.1103/PhysRevLett.109.234801
14. Qin C, Zhang H, Li S, Wang N, Li A, Fan L, et al. High efficiency laser-driven proton sources using 3d-printed micro-structure. Commun Phys-uk (2022) 5(1):124. doi:10.1038/s42005-022-00900-8
15. Gizzi LA, Cristoforetti G, Baffigi F, Brandi F, D'Arrigo G, Fazzi A, et al. Intense proton acceleration in ultrarelativistic interaction with nanochannels. Phys Rev Res (2020) 2(3):033451. doi:10.1103/PhysRevResearch.2.033451
16. Dalui M, Kundu M, Trivikram TM, Rajeev R, Ray K, Krishnamurthy M. Bacterial cells enhance laser driven ion acceleration. Sci Rep-uk (2014) 4:6002. ARTN 6002. doi:10.1038/srep06002
17. Dozières M, Petrov GM, Forestier-Colleoni P, Campbell P, Krushelnick K, Maksimchuk A, et al. Optimization of laser-nanowire target interaction to increase the proton acceleration efficiency. Plasma Phys Contr F (2019) 61(6):065016. doi:10.1088/1361-6587/ab157c
18. Khaghani D, Lobet M, Borm B, Burr L, Gartner F, Gremillet L, et al. Enhancing laser-driven proton acceleration by using micro-pillar arrays at high drive energy. Sci Rep (2017) 7(1):11366. Epub 2017/09/14. doi:10.1038/s41598-017-11589-z
19. Vallieres S, Salvadori M, Permogorov A, Cantono G, Svendsen K, Chen Z, et al. Enhanced laser-driven proton acceleration using nanowire targets. Sci Rep (2021) 11(1):2226. Epub 2021/01/28. doi:10.1038/s41598-020-80392-0
20. Feng B, Ji LL, Shen BF, Geng XS, Guo Z, Yu Q, et al. Effects of micro-structures on laser-proton acceleration. Phys Plasmas (2018) 25(10):103109. doi:10.1063/1.5037496
21. Curtis A, Hollinger R, Calvi C, Wang S, Huanyu S, Wang Y, et al. Ion acceleration and D-D fusion neutron generation in relativistically transparent deuterated nanowire arrays. Phys Rev Res (2021) 3(4):043181. doi:10.1103/PhysRevResearch.3.043181
22. Cristoforetti G, Londrillo P, Singh PK, Baffigi F, D'Arrigo G, Lad AD, et al. Transition from coherent to stochastic electron heating in ultrashort relativistic laser interaction with structured targets. Sci Rep (2017) 7(1):1479. Epub 2017/05/05. doi:10.1038/s41598-017-01677-5
23. Jiang S, Ji LL, Audesirk H, George KM, Snyder J, Krygier A, et al. Microengineering laser plasma interactions at relativistic intensities. Phys Rev Lett (2016) 116(8):085002. Epub 2016/03/12. doi:10.1103/PhysRevLett.116.085002
24. Moreau A, Hollinger R, Calvi C, Wang S, Wang Y, Capeluto MG, et al. Enhanced electron acceleration in aligned nanowire arrays irradiated at highly relativistic intensities. Plasma Phys Contr F (2020) 62(1):014013. doi:10.1088/1361-6587/ab4d0c
25. Shou Y, Kong D, Wang P, Mei Z, Cao Z, Pan Z, et al. High-efficiency water-window X-ray generation from nanowire array targets irradiated with femtosecond laser pulses. Opt Express (2021) 29(4):5427–36. Epub 2021/03/18. doi:10.1364/OE.417512
26. Purvis MA, Shlyaptsev VN, Hollinger R, Bargsten C, Pukhov A, Prieto A, et al. Relativistic plasma nanophotonics for ultrahigh energy density physics. Nat Photon (2013) 7(10):796–800. doi:10.1038/nphoton.2013.217
27. Jiang S, Link A, Canning D, Fooks JA, Kempler PA, Kerr S, et al. Enhancing positron production using front surface target structures. Appl Phys Lett (2021) 118(9):094101. doi:10.1063/5.0038222
28. Pukhov A, Sheng ZM, Meyer-ter-Vehn J. Particle acceleration in relativistic laser channels. Phys Plasmas (1999) 6(7):2847–54. doi:10.1063/1.873242
29. Curtis A, Calvi C, Tinsley J, Hollinger R, Kaymak V, Pukhov A, et al. Micro-scale fusion in dense relativistic nanowire array plasmas. Nat Commun (2018) 9(1):1077. Epub 2018/03/16. doi:10.1038/s41467-018-03445-z
30. Ceccotti T, Levy A, Popescu H, Reau F, D'Oliveira P, Monot P, et al. Proton acceleration with high-intensity ultrahigh-contrast laser pulses. Phys Rev Lett (2007) 99(18):185002. Epub 2007/11/13. doi:10.1103/PhysRevLett.99.185002
31. Fourmaux S, Buffechoux S, Albertazzi B, Capelli D, Lévy A, Gnedyuk S, et al. Investigation of laser-driven proton acceleration using ultra-short, ultra-intense laser pulses. Phys Plasmas (2013) 20(1):013110. doi:10.1063/1.4789748
32. Prasad R, Andreev AA, Ter-Avetisyan S, Doria D, Quinn KE, Romagnani L, et al. Fast ion acceleration from thin foils irradiated by ultra-high intensity, ultra-high contrast laser pulses. Appl Phys Lett (2011) 99(12):121504. doi:10.1063/1.3643133
33. Bychenkov VY, Singh PK, Ahmed H, Kakolee KF, Scullion C, Jeong TW, et al. Ion acceleration in electrostatic field of charged cavity created by ultra-short laser pulses of 1020–1021 W/Cm2. Phys Plasmas (2017) 24(1):010704. doi:10.1063/1.4975082
34. Lu X, Zhang H, Li J, Leng Y. Reducing temporal pedestal in a Ti:sapphire chirped-pulse amplification system by using a stretcher based on two concave mirrors. Opt Lett (2021) 46(21):5320–3. Epub 2021/11/02. doi:10.1364/OL.435145
35. Wang X, Han G-R. Fabrication and characterization of anodic Aluminum Oxide template. Microelectronic Eng (2003) 66(1-4):166–70. doi:10.1016/s0167-9317(03)00042-x
36. Mancic A, Fuchs J, Antici P, Gaillard SA, Audebert P. Absolute calibration of photostimulable image plate detectors used as (0.5-20 Mev) high-energy proton detectors. Rev Sci Instrum (2008) 79(7):073301. Epub 2008/08/07. doi:10.1063/1.2949388
37. Kemp AJ, Wilks SC, Hartouni EP, Grim G. Generating kev ion distributions for nuclear reactions at near solid-density using intense short-pulse lasers. Nat Commun (2019) 10(1):4156. Epub 2019/09/15. doi:10.1038/s41467-019-12076-x
38. Schnürer M, Andreev AA, Steinke S, Sokollik T, Paasch-Colberg T, Nickles PV, et al. Comparison of femtosecond laser-driven proton acceleration using nanometer and micrometer thick target foils. Laser Part Beams (2011) 29(4):437–46. doi:10.1017/s0263034611000553
39. Derouillat J, Beck A, Pérez F, Vinci T, Chiaramello M, Grassi A, et al. Smilei: A collaborative, open-source, multi-purpose particle-in-cell code for plasma simulation. Comput Phys Commun (2018) 222:351–73. doi:10.1016/j.cpc.2017.09.024
40. Andreev AA, Steinke S, Sokollik T, Schnürer M, Avetsiyan ST, Platonov KY, et al. Optimal ion acceleration from ultrathin foils irradiated by a profiled laser pulse of relativistic intensity. Phys Plasmas (2009) 16(1):013103. doi:10.1063/1.3054528
41. Gitomer SJ, Jones RD, Begay F, Ehler AW, Kephart JF, Kristal R. Fast ions and hot electrons in the laser–plasma interaction. Phys Fluids (1986) 29(8):2679. doi:10.1063/1.865510
Keywords: laser-proton acceleration, micro-wire structure, high laser-proton energy coupling efficiency, high energy density plasma, laser-induced nuclear fusion
Citation: Fan L, Xu T, Wang Q, Xu J, Zhang G, Wang P, Fu C, Ma Z, Deng X, Ma Y, Li S, Lu X, Li J, Xu R, Wang C, Liang X, Leng Y, Shen B, Ji L and Li R (2023) Enhanced laser-driven backward proton acceleration using micro-wire array targets. Front. Phys. 11:1167927. doi: 10.3389/fphy.2023.1167927
Received: 17 February 2023; Accepted: 12 June 2023;
Published: 22 June 2023.
Edited by:
Marco La Cognata, Laboratori Nazionali del Sud (INFN), ItalyReviewed by:
Giovanni Luca Guardo, Laboratori Nazionali del Sud (INFN), ItalyZhao Yongtao, Xi’an Jiaotong University, China
Copyright © 2023 Fan, Xu, Wang, Xu, Zhang, Wang, Fu, Ma, Deng, Ma, Li, Lu, Li, Xu, Wang, Liang, Leng, Shen, Ji and Li. This is an open-access article distributed under the terms of the Creative Commons Attribution License (CC BY). The use, distribution or reproduction in other forums is permitted, provided the original author(s) and the copyright owner(s) are credited and that the original publication in this journal is cited, in accordance with accepted academic practice. No use, distribution or reproduction is permitted which does not comply with these terms.
*Correspondence: Tongjun Xu, tjxu@siom.ac.cn; Guoqiang Zhang, zhangguoqiang@sari.ac.cn; Liangliang Ji, jill@siom.ac.cn